- 1Duke Molecular Physiology Institute, Duke University Medical Center, Durham, NC, United States
- 2Department of Wildland Resources, Utah State University, Logan, UT, United States
- 3Northern Great Plains Research Laboratory, United States Department of Agriculture-Agricultural Research Service (USDA)-Agricultural Research Service, Mandan, ND, United States
While commission reports and nutritional guidelines raise concerns about the effects of consuming red meat on human health, the impacts of how livestock are raised and finished on consumer health are generally ignored. Meat and milk, irrespective of rearing practices, provide many essential nutrients including bioavailable protein, zinc, iron, selenium, calcium, and/or B12. Emerging data indicate that when livestock are eating a diverse array of plants on pasture, additional health-promoting phytonutrients—terpenoids, phenols, carotenoids, and anti-oxidants—become concentrated in their meat and milk. Several phytochemicals found in grass-fed meat and milk are in quantities comparable to those found in plant foods known to have anti-inflammatory, anti-carcinogenic, and cardioprotective effects. As meat and milk are often not considered as sources of phytochemicals, their presence has remained largely underappreciated in discussions of nutritional differences between feedlot-fed (grain-fed) and pasture-finished (grass-fed) meat and dairy, which have predominantly centered around the ω-3 fatty acids and conjugated linoleic acid. Grazing livestock on plant-species diverse pastures concentrates a wider variety and higher amounts of phytochemicals in meat and milk compared to grazing monoculture pastures, while phytochemicals are further reduced or absent in meat and milk of grain-fed animals. The co-evolution of plants and herbivores has led to plants/crops being more productive when grazed in accordance with agroecological principles. The increased phytochemical richness of productive vegetation has potential to improve the health of animals and upscale these nutrients to also benefit human health. Several studies have found increased anti-oxidant activity in meat and milk of grass-fed vs. grain-fed animals. Only a handful of studies have investigated the effects of grass-fed meat and dairy consumption on human health and show potential for anti-inflammatory effects and improved lipoprotein profiles. However, current knowledge does not allow for direct linking of livestock production practices to human health. Future research should systematically assess linkages between the phytochemical richness of livestock diets, the nutrient density of animal foods, and subsequent effects on human metabolic health. This is important given current societal concerns about red meat consumption and human health. Addressing this research gap will require greater collaborative efforts from the fields of agriculture and medicine.
Introduction
Navigating discussions on red meat and human and environmental health are challenging. On the one hand, reports such as those by the EAT-Lancet Commission asks consumers to embrace near plant-exclusive diets to reduce our impacts on planetary health (Willett et al., 2019). On the other hand, reports such as those by the United Nations Intergovernmental Panel on Climate Change (IPCC, 2019) suggest a critical role for sustainable livestock production systems in climate change mitigation by integrating tree, crop, and livestock production systems, while ensuring global food security and nutrient adequacy via consumption of moderate amounts of animal foods.
Meanwhile in the field of human nutrition, a wealth of epidemiological data associate animal food consumption, particularly red meat, with increased risk of cancer (Chan et al., 2011), cardiovascular disease (Zhong et al., 2020), obesity (Wang and Beydoun, 2009), and diabetes (Micha et al., 2012). This has led to widescale public health recommendations by the American Heart Association (Arnett et al., 2019), the World Health Organization (Bouvard et al., 2015), and the Dietary Guidelines for Americans (USDA, 2015) to reduce red meat consumption in an effort to halt metabolic disease. However, these recommendations have been challenged by the NutriRECS consortium, as scrutinizing the data with the GRADE (Grading of Recommendations, Assessment, Development, and Evaluations) system resulted in considerable uncertainty regarding the robustness of evidence associating consumption of red meat with increased risk of metabolic disease (Johnston et al., 2019).
While public health experts and climate scientists argue about whether we should rely on livestock production systems to meet the nutritional demands of a growing global population while tackling climate change, those who produce our food—the “average” farmer—are struggling financially (Wiggins et al., 2010). To make a decent living, farmers must balance trade-offs between land ecology, animal welfare, and profitability, which in modern-day farming practices are not necessarily in agreement with each other. High costs for inputs, but low income from farming, has created depression and suicide rates well-above national averages amongst farmers in the US, New Zealand, Australia, and Europe (Klingelschmidt et al., 2018).
Yet some farmers have costs of production much less than the national average and are able to cut costs, and thereby increase the profitability of their operations (Provenza, 2008). Increased consumer demand for (local) grass-fed meat and milk have encouraged a number of producers to implement farming practices many describe as regenerative agriculture (i.e., farming in harmony with agroecological principles). As some farmers have learned, they are able to cut costs and increase profits by focusing on reducing inputs such as water, fertilizer, concentrate feeds, and herbicides; by raising multiple species of livestock on diverse assemblages of cover crops and/or perennial plants; and by selling their meat and milk directly to consumers (Massey, 2017; Brown, 2018).
Pasture-based grazing systems, when managed in ways that mimic natural ecosystems, can improve plant diversity (Teague, 2018), soil carbon levels (Allard et al., 2007; Stanley et al., 2018), ecosystem function (Krausman et al., 2009; Teague and Kreuter, 2020), and water retention and quality of fresh water systems (Park et al., 2017). As the IPCC (2019) notes, Earth's health depends upon plant diversity and abundance, which can be improved by managing the grazing behavior of livestock when done in concert with agroecological principles (i.e., that mimic natural processes). That should come as no surprise in the light of plant-herbivore coevolution, which have evolved to form complex reciprocal relationships over millions of years.
The constant “arms race” between plants and herbivores has resulted in an extraordinary diversity of phytochemicals produced by plants (Burkepile and Parker, 2017). In turn, many of these plant phytochemicals are concentrated in the meat and milk of livestock grazing these plants (Børge et al., 2016; Delgadillo-Puga et al., 2019; Prache et al., 2020); their presence may act synergistically to enhance human health (Barabási et al., 2020). Importantly, the presence of these phytochemicals in pasture-raised animal products remains largely underappreciated in discussions of nutritional differences between grain-fed and pasture-raised (grass-fed) meat and dairy, which have predominantly been centered around the ω-3 fatty acids and CLA (Provenza et al., 2019). In this review, we discuss the information currently available on the wide range on phytochemicals found in grass-fed meat and dairy products and evaluate their potential health effects.
Why Becoming Locally Adapted Matters
Natural landscapes are diverse mixtures of plants that occur in patches reflecting history of use in concert with particular soil, precipitation, and temperature regimes. Plants are nutrition centers and pharmacies with vast arrays of primary (e.g., protein, fiber, carbohydrates, fats, vitamins, and minerals) and secondary compounds (e.g., phenols, terpenoids, anti-oxidants, organic acids, and other phytochemicals) useful in both animal (Craig, 1999; Poutaraud et al., 2017) and human health (Kris-Etherton et al., 2002; Chadwick et al., 2013; Kim, 2016).
Of roughly 200,000 species of wild plants on earth, only a few thousand are eaten by humans, just a few hundred of these have been domesticated, and only a dozen account for over 80% of the current annual production of all crops (FAO, 2010). By focusing on a few species, people transformed the diverse world of plants into a manageable domain that generally met needs for nutrients, mainly energy, while limiting over-ingestion of toxins (Johns, 1994). In so doing, however, we narrowed the genetic basis of crop production to just a few plants, relatively productive in a broad range of environments, rather than broadening the range of plants that are valuable in local environments (Shelef et al., 2017). We have also discovered only a fraction of the plant mixtures useful in nutrition and health (Etkin, 2000) and we have simplified agricultural systems in ways that are having alarming consequences on the health of people and the planet (Provenza, 2018). Though often successful in the short term, “simplifying” ecosystems can lead to ruinous long-term impacts, as shown in marine, forest, and rangeland systems (Gunderson et al., 1995). By maximizing the output of one component of a system, we inevitably hasten the demise of ecosystems.
The United Nations Commission on Genetic Resources for Food and Agriculture has declared the loss of biodiversity as one of the major threats to the productivity and resilience of food production systems (Pilling et al., 2020). Biodiversity amongst soil microorganisms, plants, and animals, which have co-evolved to form complex symbiotic relationships over millions of years, are essential to maintain soil health and sustainable agroecosystems (Coleman and Whitman, 2005; De Faccio Carvalho et al., 2010). The structural and functional diversity inherent in natural systems increases productivity of plant and animal species, and enhances system resilience. Studies of natural systems highlight the benefits of plant and animal biodiversity for reducing inter-annual variability in production and minimizing risk of large-scale catastrophic events, such as wildfire and outbreaks of diseases and pests (Gunderson et al., 1995; Provenza et al., 2007). Diversity in terms of nutrition also increases the range of options available for both animals and humans to nourish themselves and medicate prophylactically.
Along with reductions in per capita meat consumption in industrialized nations (Godfray et al., 2018), meat consumption will arguably need to increase in developing nations to meet basic needs for protein, essential fatty acids, and micronutrients (Adesogan et al., 2019). It was recently estimated that animal-sourced food consumption at 1/3 of total energy intake (i.e., a 1:2 energy intake ratio of animal to plant foods) will provide nutritionally adequate diets for most of the global population (Nordhagen et al., 2020), a target currently not met in the majority of low-to-middle income countries (FAO, 2020). By including complementary nutrient-dense plant foods (Eshel et al., 2019), and depending on intra-individual differences in nutrient metabolism (Brenna, 2002; Stover and Caudill, 2008; Tang, 2010; Engelken et al., 2014), even lower amounts of animal sources foods may be sufficient to achieve nutrient adequacy in certain individuals within the population. That is more feasible in high-income than low-income countries as a result of food availability (FAO, 2020).
Beyond meeting basic nutrient requirements, people in low-income countries often depend on livestock rearing not just for food, but for their entire livelihood; at the household level, livestock contribute 68% of income in low- to middle-income households (FAO, 2009). For the poorest, grazing livestock is an effective way to reduce poverty (Omamo et al., 2006) from the sale of excess foodstuff, fiber, and waste products, especially when livestock are managed in ways that sustain the natural resource base (e.g., increase soil organic carbon content, reduce soil erosion, and integrate crop-livestock systems) (IPCC, 2019). Importantly, 3.4 billion hectares (70% of current agricultural land) of land will support livestock and wild animals, but not crop production (FAOSTAT, 2020). That is significant because the majority of those lands are in developing nations and are home to billions of people who depend on livestock grazing for their livelihood.
As ecological, economic, and human health concerns mount—soil, water, and climate change; animal welfare; and red meat consumption and human health—demand for livestock reared on pasture will further increase in both developing and developed nations. For example, the US grass-fed beef market increased from $17 million in retail sales in 2012 to $480 million in 2019, and this trend is expected to continue in the years ahead (Nielsen, 2020). Moreover, the organic dairy market is expected to register a compound annual growth rate of 10% in the next 5 years (Kumar and Deshmukh, 2019). The challenges and opportunities are to create grazing-based livestock-production systems based on phytochemically diverse forages for specific ecoregions at temporal and spatial scales that enhance livestock production, ecological services, and animal and human health (Gregorini et al., 2017; Provenza et al., 2019).
While understanding animal adaptations to landscapes has always been an important aspect of nutritional ecology (Demment and Van Soest, 1985), until recently land managers have not attempted to put these ideas into practice on a wide-scale. We often consider cattle to be grass eaters and sheep to be forb eaters; however, they can thrive under a wide range of conditions, including shrub-dominated areas in the arid southwest US (Provenza and Balph, 1990), high-altitude pastures in the Alps (Verrier et al., 2005), and the Amazon rainforest (Loker, 1994), provided they have been selected anatomically, physiologically, and behaviorally to survive on their own in the landscapes they inhabit, and are compatible with wildlife inhabiting these environments (Provenza, 2008).
Offering locally-adapted animals choices on pastures and rangelands also allows each individual to meet its needs for nutrients and to regulate its intake of secondary compounds by mixing foods in ways that work for that individual (Provenza et al., 2003). Cattle, sheep, and goats eat more and perform better when they are offered a wide variety of plants that contain secondary compounds (Provenza et al., 2007). Variety is so important that bodies have built-in mechanisms to ensure animals eat a variety of foods and forage in different locations to satiate and meet nutritional requirements (Bailey et al., 1996; Provenza, 1996). Thus, variety not only enables individuality, it also greatly increases the likelihood of providing cells with the vast arrays of primary and secondary compounds essential for their nutrition and health. While we often do not think of animals as intelligent beings; animals, unlike humans, do not have to be told what to eat and nurture themselves prophylactically—to prevent disease—and medicinally—to treat disease (Provenza, 2008).
Livestock are intelligent beings (Marino and Allen, 2017); they possess most of the mental, emotional, and behavioral traits we identify in humans, and by nurturing livestock we can nurture ourselves (Provenza et al., 2019). During the Green Revolution (1950-60s), agricultural systems largely moved away from integrated multi-species livestock-crop systems toward farming systems where livestock are separated from plant farming, and finished (cattle and sheep) or raised almost exclusively (poultry and pigs) in concentrated operations where animals are fed total mixed rations. Depending on practices, confined feeding systems can thwart the animals' ability to self-select their own diet and express natural behavior, which can adversely affect their welfare and health.
For example, Carrillo et al. (2016) found increased blood glucose and cortisol levels in feedlot-finished vs. pasture-raised cattle, which indicates impaired metabolic health and increased stress in the feedlot-fed animals. Metabolomic and gene expression analyses also revealed mitochondrial dysfunction and impaired oxidative phosphorylation in muscle tissue of feedlot-finished cattle. These findings were corroborated by a recent report demonstrating that meat of grass-fed animals displays a phenotype of improved oxidative metabolism compared to meat from feedlot-finished animals (Apaoblaza et al., 2020). This is consistent with studies of lambs, who display similar elevations in blood cortisol and behavioral changes indicative of stress and fear when fed total-mixed rations, formulated for the “average lamb,” compared to having a broader dietary choice (Catanese et al., 2013).
Importantly, the metabolic phenotype of the feedlot-finished animals described in the work by Carrillo et al. (2016) and Apaoblaza et al. (2020) shows similarity with the human phenotype of metabolic disease, which is also characterized by muscle mitochondrial dysfunction (Nisoli et al., 2007), increased oxidative stress (Whaley-Connell et al., 2011), and elevated blood glucose (Grundy et al., 2004) and cortisol (Rosmond, 2005). In contrast, the greater mitochondrial oxidative enzyme content in pasture-raised animals (Apaoblaza et al., 2020) can be considered that of a healthy athletic phenotype. Certainly, animal health issues can also arise in ill-managed pasture-based systems. While causality cannot be inferred from these data, the link between consuming meat and dairy products from animals—that display varying degrees of metabolic health—and the subsequent effects on human metabolic health requires further examination.
Linking Livestock Systems to Human Health
Several studies associate red meat, and to a lesser extent dairy, with an increased risk of cardiovascular disease (Kaluza et al., 2012; Ding et al., 2019), cancer (Ganmaa et al., 2002; Chan et al., 2011; Fraser et al., 2020), type II diabetes (Pan et al., 2011), and early mortality (Schwingshackl et al., 2017b; Zheng et al., 2019). However, associations between red meat and increased disease risk are not supported by several other studies, especially when dietary quality is high (i.e., diets rich in fruits/vegetables and low in ultra-processed foods) (Schulze et al., 2003; Kappeler et al., 2013; Grosso et al., 2017; Maximova et al., 2020). In the case of dairy, higher intakes are often also found to be neutral or even protective (Aune et al., 2012; Guo et al., 2017; Schwingshackl et al., 2017a). Regardless of the directionality of associations and potential nuances of residual confounding in associative data, currently available epidemiological data do not differentiate among ways that livestock are raised and finished (on pasture or feedlots) nor do epidemiological studies often discriminate different types of red meat (e.g., beef, pork, or lamb) and dairy (e.g., cow, goat, or sheep) that people consume (Provenza et al., 2019).
To determine whether livestock rearing practices modulate health associations, we encourage epidemiological studies to include questions regarding sourcing of meat and dairy (e.g., grass-fed, pasture-raised, organic, “conventional” [no designation on package] etc.) on self-reported dietary recalls and Food Frequency Questionnaires. A potential caveat is that those who buy more pasture-raised meat and dairy are more health-conscious to begin with (Ziehl et al., 2005), highlighting a need to account for both lifestyles and individual diets in studies comparing health associations with meat consumption from different livestock production systems (e.g., pasture-raised, grass-fed vs. grain-fed).
Meat and milk consumption, irrespective of rearing practices, substantially contribute to many essential nutrients in the human diet including protein, zinc, selenium, iron, phosphorus, calcium, and vitamins B12 and D (Phillips et al., 2015; De Gavelle et al., 2018). While little difference exists in total protein content between pasture-raised and feedlot-finished meat and dairy (Duckett et al., 2009; Schönfeldt et al., 2012), differences do exist in vitamins and trace minerals. For example, Duckett et al. (2009) compared riboflavin and thiamine in grass-finished vs. grain-finished beef, and found nearly 2-fold higher riboflavin concentrations and 3-fold higher thiamine concentrations in grass-finished beef. Pasture-raised meat and dairy also have more favorable fatty acid compositions compared to their feedlot-fed counterparts (Daley et al., 2010; Benbrook et al., 2018). Pasture-raised meat and dairy is generally lower in saturated fat and cholesterol, and contains more conjugated linoleic acid (CLA) and ω-3 fatty acids compared to feedlot-finished counterparts (Bergamo et al., 2003; Daley et al., 2010; Villeneuve et al., 2013; Coppa et al., 2019). It is often stated that ω-3 fatty acids are present in such modest amounts in pasture-raised beef that any difference between pasture-raised vs. feedlot-fed beef is not of biological relevance. This is arguably the case for the ω-3 fatty acids docosahexaenoic acid (DHA) and eicosapentaenoic acid (EPA), which have been studied extensively for their ability to lower metabolic disease risk (Kris-Etherton et al., 2003; Calder, 2010). This notion, however, fails to take into account the abundance of the ω-3 fatty acid docosapentaenoic acid (DPA) in pasture-raised beef, which can effectively be converted to EPA in vivo in humans (Miller et al., 2013), and may exert anti-inflammatory effects on its own (Dalli et al., 2013). Eating pasture-raised beef increased blood levels of DPA and EPA concentrations of humans, while no such effects were observed with feedlot-finished beef (McAfee et al., 2011).
Dairy and meat from pasture-raised ruminants are also rich sources of conjugated linoleic acids (CLA). CLA refers to a family of geometric and positional isomers of 18-carbon linoleic acids that are produced by bacterial biohydrogenation of forage in the rumen or through subsequent δ-9-desaturase enzymatic conversion of trans-vaccenic acid (TVA) in the mammary gland and/or adipose tissue (Jahreis et al., 1997). While humans can also produce certain isomers of CLA in vivo through δ-9-desaturase conversion of TVA (Kuhnt et al., 2006), this conversion is so low that meat and milk represent the main sources of CLA for humans (Adlof et al., 2000).
CLA is predominantly studied for its anti-carcinogenic and anti-adipogenic properties. For instance, consumption of CLA-rich butter reduced cancer proliferation in animal models of breast cancer (Ip et al., 1999). In addition, dietary intake of CLA and serum concentrations of CLA are inversely related to risk of breast cancer and colorectal cancer in some (Aro et al., 2000; Larsson et al., 2005), but not all (Norris et al., 2009), population-based studies. Several studies find that the CLA content is 1.5 and 3 times times higher in pasture-raised meat and dairy, respectively, than grain-fed products (Dhiman et al., 1999; Daley et al., 2010; Benbrook et al., 2018), an outcome believed to be due to a more favorable rumen pH as a result of forage- as opposed to grain-feeding (Bessa et al., 2000). Unsurprisingly, consuming pasture-raised animal products elevates serum CLA concentrations in humans compared to grain-fed animals (Ritzenthaler et al., 2005; Brown et al., 2011).
While improved fatty acid ratios (ω-3: ω-6) and CLA have been the predominant focus in comparisons of pasture-raised, grass-fed vs. grain-fed meat and milk, emerging data indicate that when livestock are eating a diverse array of plants on pasture, many plant phytochemicals are also concentrated in their meat and milk (Prache et al., 2005; Carrillo et al., 2016). This is noteworthy as phytochemicals are often considered to occur only in plant foods.
Phytochemicals in Meat and Milk
Terpenoids
Terpenoids—monoterpenes, diterpenes, and sesquiterpenes—are a large and diverse class of phytochemicals studied extensively for their anti-inflammatory, anti-oxidant, anti-viral, and anti-carcinogenic properties (Zhang et al., 2005; Merfort, 2011; Chadwick et al., 2013). The presence of terpenoids in animal foods is directly related to the terpenoid composition of the animal's diet (Vialloninsta et al., 2000; Bugaud et al., 2001; Priolo et al., 2003). Animals grazing more botanically diverse pastures accumulate both higher amounts and a wider variety of terpenoids (and other phytochemicals) in their meat and milk compared to animals grazing non-diverse (i.e., monoculture) pastures, while concentrations of phytochemicals are further reduced—and often remain undetected—in the meat and milk of animals fed grain-based diets in feedlots (Figure 1).
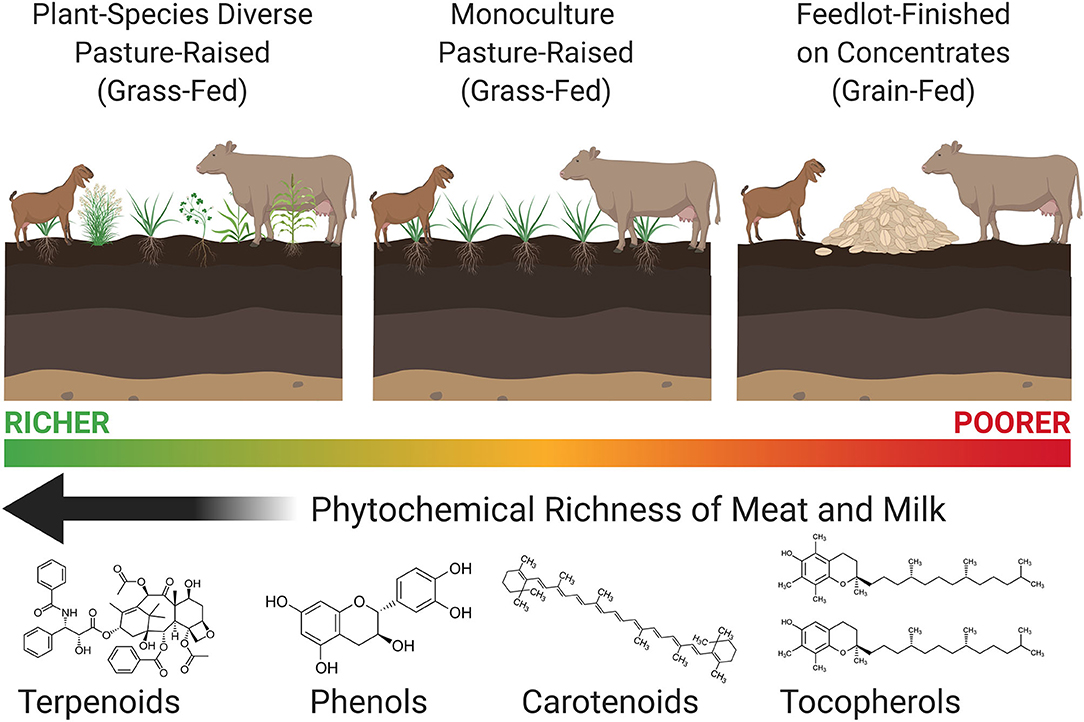
Figure 1. The effects of livestock production systems on the phytochemical richness—terpenoids, phenols, carotenoids, and tocopherols—of meat and milk. Grazing livestock on plant-species diverse pastures concentrates a wider variety of phytochemicals in their meat and milk compared to animals grazing non-diverse pastures, while phytochemicals are further reduced and often remain absent in meat and milk from animals fed grain-based concentrates in feedlots. Created with BioRender.com.
Milk obtained from cattle grazing diversified forages contained 6 to 23 times more monoterpenes (α-thujene, camphene, o-cymene) and sesquiterpenes (α-copaene, ß-caryophyllene) than milk obtained from animals fed concentrates (Martin et al., 2005). Similarly, Agabriel et al. (2007) found that terpenes—such as α-copaene (anti-oxidant), β-bourbonene (anti-tumor, apoptosis inducer), β-pinene (anti-inflammatory, anti-oxidant, anti-tumor), β-Elemene (anti-inflammatory, anti-tumor), and sabinene (anti-inflammatory, anti-oxidant)—were higher in milk from pasture-raised animals with access to more forage diversity compared to animals fed grain-based diets. Likewise, Børge et al. (2016) found that δ-3 Carene, α+β-pinene, α-thujene, ß-citronellene, and sabinene concentrations (anti-inflammatory, anti-bacterial, anti-oxidant, and/or anti-carcinogenic) were collectively 5-fold higher in cream produced from animals raised on diversified pasture compared to cream from animals fed concentrates.
Amongst pasture-based systems, greater botanical diversity of forage generally results in higher terpenoid concentrations in meat and milk. For example, goats grazing a wide variety grasses, legumes, and forbs concentrated 5-fold more terpenoids in their milk compared to goats consuming a limited number of grasses (alfalfa, perennial rye grass, and orchard grass) (Fedele et al., 2007). Similar findings were made by Martin et al. (2005) who found that milk samples from cattle grazing diversified mountain pastures were enriched in terpenoids compared to cattle grazing a ryegrass monoculture. While the presence of terpenoids have predominantly been studied in dairy systems, higher concentrations of terpenoids have also been found in the meat of grass-fed cattle (Larick et al., 1987) and lambs (Priolo et al., 2003) compared to their grain-finished counterparts (Table 1).
Besides differences in pasture diversity, seasonality also impacts the terpenoid content of milk with highest concentrations observed during the summer, compared to the fall and spring, while concentrations are lowest during the winter (Agabriel et al., 2007; Chion et al., 2010; Valdivielso et al., 2017). These findings can be ascribed to the differences in cattle diets (fresh pasture vs. hay) (Valdivielso et al., 2017) and/or seasonal differences in terpenoid content of grazed plants (Mariaca et al., 1997). These differences can be substantial, as demonstrated by Valdivielso et al. (2017), who found that terpenoids were 5-fold higher in the summer compared to the winter, and 2-fold higher in the summer compared to the spring. Even during winter, when terpenoids concentrations in pasture-fed milk were lowest, concentrations remained 2-fold higher for pasture-fed compared to grain-fed animals, which obviously experience little seasonality due to largely standardized total-mixed ration feeding throughout the year (Agabriel et al., 2007).
Phenols
Phenols in plants exert strong in vivo anti-oxidative and anti-inflammatory effects in both animals (Poutaraud et al., 2017) and humans (Zhang and Tsao, 2016). Phenols consist of one (phenolic acids) or more (polyphenols) aromatic rings with attached hydroxyl groups as part of their structures (Lewandowska et al., 2013). Therapeutic benefits of phenols include protection again various cancers (Kampa et al., 2007; Zhou et al., 2016), hepatic disorders (Saha et al., 2019), cardiovascular disease (Khurana et al., 2013), neurodegenerative diseases (Vauzour, 2012), type 2 diabetes (Guasch-Ferré et al., 2017), obesity (Wang et al., 2014), improved immune function (Ding et al., 2018), and gut microbial composition (Cardona et al., 2013).
Similar to terpenoids, the presence of phenols in milk is directly related to the phenolic composition in the diet (De Feo et al., 2006; Besle et al., 2010; Di Trana et al., 2015), with higher concentrations observed during summer compared to winter (Cabiddu et al., 2019), and higher concentrations in meat and milk from animals fed a botanically diverse diet on pasture compared to animals raised on monoculture pastures, with lowest concentrations found in animals fed grain-based concentrates (Table 1). For example, flavonoid and caffeoylquinic acid content is 6-fold higher in milk from cattle raised on botanically-diverse mountain pastures compared to cattle raised on monoculture grasses, and is largely undetected in milk from animals fed concentrates in confinement (Besle et al., 2004, 2010). In these studies, concentrations of phenols showed a clear stepwise increase as botanical diversity of forage increased (grassland pasture > grassland hay > ryegrass hay > ryegrass silage > concentrates). Likewise, Delgadillo-Puga et al. (2019) found that catechin, chlorogenic acid, gallic acid, and ferulic acid were present only in the milk of goats grazed on pasture and were undetectable when goats were fed concentrates in confinement. Finally, concentrations of equol, formononetin (both isoflavones), and enterolactone (a lignan) are higher in milk from cattle grazed on pasture than in milk from feedlots (Hoikkala et al., 2007; Adler et al., 2015). Higher equol and/or enterolactone intakes are associated with a reduced risk of osteoporosis, prostate, and breast cancer, as well as lower circulating levels of low-density lipoprotein cholesterol and C-reactive protein (Jackson et al., 2011; Rodríguez-García et al., 2019).
Pasteurization is another factor impacting the polyphenol content of dairy. For example, Cuchillo-Hilario et al. (2009) found that the polyphenol content in pasture-raised goat cheese was 3-fold higher in raw milk cheese than when the milk was pasteurized prior to making cheese. While pasteurization is the norm in the US, consumption of raw dairy is more accepted in other parts of the world, including several European and African countries (Mattiello et al., 2018; Baars, 2019; Meunier-Goddik and Waite-Cusic, 2019). For example, in the Netherlands, Gouda farmhouse cheeses are required by law to conform to traditional production methods, which prohibit pasteurization (Fischer Boel, 2009). Similarly, French artisanal cheeses are often made from raw milk and are considered superior to pasteurized cheeses. Besides enhancing health, phytochemicals also enhance flavor (Clarke et al., 2020), which can become compromised with heat treatment. Proper animal husbandry and hygiene practices on farms would enable farmers to obtain raw milk of high microbiological quality (D'Amico and Donnelly, 2010; Janštová et al., 2011; Giacometti et al., 2013; Baars, 2019). Nevertheless, however small the risk of pathogenic presence may be— <0.3% in aforementioned studies, generally limited to single farms—zero-risk is not possible and consuming raw milk can have serious adverse health effects (O'Callaghan et al., 2019). Whether such small risks are acceptable in food systems and whether the consumption of raw dairy from pasture-based systems (milk from concentrated feeding operations is generally not sold raw) should be left to individual choice is beyond the discussion of our work (for a nuanced discussion of the topic, see Baars, 2019). Cooking meat also reduces polyphenol and terpenoid contents by 25–60% (King et al., 1993, 1995; Kozová et al., 2009; Dadáková et al., 2011), with higher heat preparations (e.g., grilling and roasting) resulting in higher reductions compared to lower heat preparations (e.g., stewing and “sous vide”) (Kozová et al., 2009; Dadáková et al., 2011).
The contribution of phytochemicals from pasture-raised meat and milk to overall dietary intake should not be underestimated. While total phenolic levels (expressed as gallic acid equivalents) in fruits and vegetables (Deng et al., 2013) are generally 5 to 20-fold higher compared to pasture-raised meat and milk (López-Andrés et al., 2013; Chen et al., 2015; Cabiddu et al., 2019; Delgadillo-Puga et al., 2019), various individual phytochemicals are abundant in pasture-raised meat and milk. For example, the amount of chlorogenic acid (12.3 mg/100 g) in pasture-raised milk (Delgadillo-Puga et al., 2019) may be on par or higher than several vegetables and fruits such broccoli (5.5 mg/100 g), cowpea (0.7 mg/100 g), and tomatoes (8 mg/100 g) (Deng et al., 2013; Arnaud, 2016). Furthermore, López-Andrés et al. (2013) found total phenolic levels in pasture-fed lamb liver to be 6.6 mg GAE/g product, which is comparable to values found in several fruits and vegetables including eggplant (6.7 mg GAE/g) and turnip (6.0 GAE/g), squash (5.0 mg GAE/g) and snap bean (5.9 mg GAE/g). Muscle meat is about 4-fold lower in phenolics compared to liver yet can still contributes meaningful amounts of phytochemicals in the human diet (Chen et al., 2015). Most commonly known for their presence in green tea, the flavonoids catechin, gallic acid, and chlorogenic acid have been extensively studied for their anti-oxidant, anti-inflammatory, and anti-carcinogenic effects (Kroes et al., 1992; Khan and Mukhtar, 2007; Verma et al., 2013). Importantly, the quantity of gallic acid (1.3 mg/100 g) and catechin (4.3 mg catechin/100 g) in diverse pasture-raised milk (Delgadillo-Puga et al., 2019) is comparable to average quantities found in some green teas (1.2 mg gallic acid/100 g; 4.3 mg catechin/100 g, respectively) (Henning et al., 2003). Similarly, the presence of quercetin and caffeic acid, which are anti-oxidants best known for their presence in onions (McAnlis et al., 1999) and coffee (Nardini et al., 2002) have also been readily detected in cheese from goats grazing botanically diverse shrubs and grasses (De Feo et al., 2006; Cuchillo-Hilario et al., 2009), albeit at 30-fold lower concentrations than what is found in onions, but similar to levels in cabbage, celery, potatoes, and several other fruits and vegetables (USDA, 2016b). We stress that these examples should not be interpreted as a meat vs. plant foods discussion nor as “evidence” that animal foods negate a need for obtaining phytochemicals from plant foods. Plant and animal foods arguably improve human health in synergistic ways (Barabási et al., 2020; Van Vliet et al., 2020). Rather these examples serve to illustrate that pasture-raised animal foods can contribute substantially to phytonutrient intake in the human diet. Whether the potential beneficial effects of consuming phytochemically-rich meat and dairy are analogous to, but distinct from, benefits attained by eating phytochemically-rich herbs, fruits, and vegetables should be assessed in future studies. Importantly, by consuming phytochemically-rich meat and milk we ingest a broad spectrum of phytonutrients from classes of plants (e.g., a wide variety of Monocotyledoneae and Dicotyledoneae) otherwise not readily consumed by humans.
Carotenoids and Tocopherols
Carotenoids are a class tetraterpenoids found abundantly in plants and fruits. β-carotene is the main isomer found in plants, however other carotenoids such as α-carotene and the xanthophylls lutein and zeaxanthin are also widely found in plants (Khoo et al., 2011; Eisenhauer et al., 2017). Carotenoids are fat soluble with β-carotene being quantitatively most abundant and responsible for the yellow tint of the fat in grass-fed beef and cow's milk (goats and sheep do not accumulate β-carotene, hence their milk is whiter) (Nozière et al., 2006; Dunne et al., 2009). Carotenoids can serve as precursors of vitamin A in humans (Haskell, 2012), and their intake is associated with a wide variety of health benefits including improved cognitive function (Grodstein et al., 2007), reduced risk of metabolic diseases such as cancer (Nishino et al., 2009), cardiovascular disease (Voutilainen et al., 2006), and diabetes (Sluijs et al., 2015), protection from age-related macular degeneration (Seddon et al., 1994), as well as more broadly being defined as having anti-oxidative and anti-inflammatory effects (Ciccone et al., 2013).
Tocopherols are a class of phenolic compounds, with vitamin E activity, best known for their anti-oxidative effects. Tocopherols exist as four structural isomers (α, β, γ, and δ) with α-tocopherol being the predominant form in both livestock (Nozière et al., 2006) and humans (Kinsella, 2007). Similar to carotenoids, tocopherols protect against cardiovascular disease (Huang et al., 2019), certain cancers (Helzlsouer et al., 2000; Das Gupta and Suh, 2016), neurocognitive decline (Mangialasche et al., 2012), and age-related macular degeneration (Delcourt et al., 1999).
Similar to terpenoids and phenols, the nature of forage also impacts carotenoid and tocopherol levels in meat and milk. Highest concentrations are found in animal grazing diverse pastures, with lower concentrations found in meat and milk from animals grazing monoculture pastures or fed dried hay (due to ultraviolet-light degradation of carotenoids after harvesting), while animals fed concentrates have the lowest concentrations of carotenoids and tocopherols (Nozière et al., 2006; Dunne et al., 2009) (Table 1). Positive curvilinear relationships were found between carotenoid intake and milk content of cows (Calderón et al., 2007) and the fat content of sheep (Dian et al., 2007). Others also found that, as the ratio of forage-to-total-mixed-rations increases, α-tocopherol, β-carotene, lutein, and/or retinol concentrations increase in a curvilinear fashion in milk (Pizzoferrato et al., 2007; La Terra et al., 2010; Salado et al., 2018).
Similar to other phytochemicals, seasonality affects carotenoid and tocopherol content in meat and milk from pasture-based systems, with higher amounts observed during the spring compared to the summer, fall, and winter (Nozière et al., 2006; Marino et al., 2012; Jain et al., 2020). For example, Nozière et al. (2006) found that β-carotene is highest in pasture-fed cow's milk during the summer, declines 1.5-fold during the fall, is 2-fold lower during the winter, and climbs back up during the spring. Similarly, Jain et al. (2020) found ~10% lower β-carotene and α-tocopherol in US grass-finished beef during the fall compared to the spring, in addition to slightly lower iron and zinc concentrations. Carotenoid content of meat experiences less seasonal variation compared to milk. That is because the transfer of phytochemicals from forage into milk occurs within days (Vialloninsta et al., 2000; Calderón et al., 2007), while the phytochemical accumulation in meat occurs slowly over the lifetime of the animal (Prache et al., 2003).
While tocopherols and carotenoids are several-fold more abundant in plant foods compared to animal foods, their presence is noteworthy as they can protect meat and milk from protein and lipid oxidation (Realini et al., 2004; Pizzoferrato et al., 2007; Gonzalez-Calvo et al., 2015), which may improve protein digestibility and amino acid availability (Lund et al., 2011), lower the formation of pro-inflammatory compounds such as aldehydes generated by cooking (Lynch et al., 2001), and increase shelf life (McDowell et al., 1996). Moreover, eating pasture-raised beef raised circulating α-tocopherol and β-carotene to 1.2 and 1.5 times higher concentrations compared to feedlot-finished beef (Tartaglione et al., 2017).
Anti-oxidant Capacity of Pasture-Raised Meat and Dairy
Oxidative stress—characterized by excessive concentrations of reactive oxygen species (ROS)—can damage proteins, DNA and RNA, and plays a major role in the development of chronic ailments such as cardiovascular (Dhalla et al., 2000) and neurodegenerative diseases (Kim et al., 2015), cancer (Reuter et al., 2010), arthritis (Quiñonez-Flores et al., 2016), diabetes (Giacco and Brownlee, 2010), and other chronic diseases (Pena-Oyarzun et al., 2018). Antioxidants act as ROS “scavengers” by preventing and repairing damage caused by ROS, thus strengthening the immune system and offering protection against developing chronic diseases (Lushchak, 2014).
The increased phytochemical richness of the diets of animals grazing botanically diverse pastures results in higher anti-oxidant capacity in meat and milk. Anti-oxidant capacity is reduced when animals forage on monocultures of grass and the lowest anti-oxidant capacity occurs in meat and milk produced from animals fed concentrates in confinement (Descalzo et al., 2005; Cuchillo-Hilario et al., 2009; Kuhnen et al., 2014; Delgadillo-Puga et al., 2019) (Table 2). For example, Delgadillo-Puga et al. (2019) found that antioxidant capacity of pasture-raised goat's milk, assessed by its ferric ion antioxidant power (FRAP) (Huang et al., 2005), was 1.5- to 2.5-fold higher than milk from goats fed concentrates. Importantly, they found that anti-oxidant capacity of the milk was strongly correlated with the presence of phenols.
Descalzo et al. (2007) found that grass-fed beef also had a 1.5-fold higher FRAP capacity than beef from grain-fed animals. Additionally, compared to grain-fed beef, grass-fed beef had a higher glutathione content and redox potential (glutathione is one of the most potent intracellular antioxidants), and superoxide dismutase activity (an enzyme providing cellular defense against ROS) (Table 2). No differences were observed for other measures of anti-oxidant status such as Trolox equivalent antioxidant capacity (TEAC), catalase (CAT), and glutathione peroxidase (GPx). In contrast, Gatellier et al. (2004) found higher CAT and GPx activity for animals fed concentrates indoors compared to pasture-grazed animals, whereas the reverse was found for SOD activity. While the reasons for these divergent findings are unclear, a potential explanation is that the concentrate-fed animals were finished on private farms for only 3 months during the winter, which may not represent “typical” feedlot environments where animals are finished on concentrates for an average of 5 months (Drouillard, 2018). As the authors point out, the findings of their study may indicate a “production system effect” rather than a diet effect. Contrary to the feedlot-finished animals, pasture-finished animals can more freely engage in natural behaviors, which could further positively impact their anti-oxidant status (Gatellier et al., 2004). Finally, López-Andrés et al. (2013) found higher anti-oxidant activity (assessed by FRAP and Folin–Ciocalteu assays) in the liver of lambs raised on pasture compared to lambs fed concentrates in confinement.
Taken together, these data suggest that pasture-raising and finishing is beneficial for both the health of the animal and its meat and milk products. It is perhaps no surprise that the two are connected: a healthier animal provides healthier meat and milk. While the phytochemical richness and anti-oxidant capacity is enhanced in grass-fed meat and dairy, especially when raised on nutrient-rich species-diverse pastures, compared to animals that fed grain-based concentrates in confinement (e.g., feedlots), the question remains: Does the increased phytochemical richness of grass-fed meat and dairy have an appreciable effect on improving human health?
The Effects of Animal Production Systems on Human Metabolic Health
The metabolic effects of consuming grass-fed meat and dairy vs. feedlot-finished counterparts have predominantly been studied for their ability to modulate inflammation and lipoprotein profiles (e.g., cholesterol and triglycerides). Low-grade systemic inflammation—characterized by elevated levels of the cytokines interleukin-6 (IL-6), tumor necrosis factor-alpha (TNF-α), and/or C-reactive protein (CRP)—participates in the development of metabolic diseases such as heart disease, cancer, type II diabetes, and arthritis (Libby, 2007). Importantly, cytokines are modulated in response to single meals (Holmer-Jensen et al., 2011), with compounding effects on the progression of metabolic disease when single meals that raise inflammation become dietary habits (Esposito and Giugliano, 2006). Modulating the inflammatory milieu by dietary choices, therefore, represents an important strategy to prevent or treat metabolic disease.
In a randomized cross-over design, Arya et al. (2010) found that eating kangaroo meat, obtained from animals foraging on native pastures, attenuated the postprandial rise in IL-6, TNF-α, and CRP compared to feedlot-finished (grain-fed) beef. A limitation of the study is that, despite all of the fat being cut off of both the beef and kangaroo steak, the kangaroo was presumably still leaner than the beef, which could have confounded the results. Another study found that daily consumption of pecorino cheese—made from sheep who foraged on diverse pastures in Tuscany, Italy—for 10 weeks reduced circulating levels of pro-inflammatory cytokines and improved erythrocyte deformability, which is indicative of improved red blood cell function (Sofi et al., 2010). Werner et al. (2013) showed similar decreases in CRP over 12-weeks with daily consumption of 39 g of butter from either mountain-raised or feedlot-fed cattle, despite a lower SFA content and improved ω-3-to-ω-6 ratio in pasture-raised butter. Total daily saturated and polyunsaturated fat intake was similar between groups, which could have washed-out any effects of the butter per se. No effect of either intervention was observed for lipid profiles, insulin sensitivity, or glucose tolerance.
Gilmore et al. (2011) found that consumption of 113g of beef, 5 times per week for 5-weeks, from cattle raised on non-diverse pasture (coastal Bermuda grass) or grain-finished in feedlots does not differentially impact inflammatory profiles (Gilmore et al., 2011). As highlighted in Tables 1 and 2, the phytochemical richness and anti-oxidant capacity is reduced in meat from animals raised on monoculture pastures compared to meat from animals with access to more forage diversity, and that could be a reason for the lack of changes in inflammatory biomarkers in this work. However, future clinical trials comparing inflammatory responses to botanically diverse diets vs. monoculture grass-fed diets vs. grain-fed meat (and dairy) are needed to test this hypothesis.
In the work of Gilmore et al. (2011), eating grain-fed beef patties also resulted in higher circulating levels of total high-density lipoprotein cholesterol (HDL-C) and triglycerides (Adams et al., 2010; Gilmore et al., 2011), but that was not observed when the group ate pasture-raised beef patties. When considering HDL-C differences by particle size, no differences were observed in large and medium HDL particle size between interventions. Levels of large HDL particles show the strongest inverse relationship with cardiovascular risk compared to other HDL particle sizes in population-based studies (Mora et al., 2007; Van Der Steeg et al., 2008). In contrast, Brown et al. (2011) found that consuming dairy and meat from pasture-fed cattle for 8 weeks did not alter lipoproteins profiles, body composition, or glucose tolerance compared to a diet comprised of products from grain-fed cattle. Fatty acid profiles were similar for SFA, MUFA, and PUFA, only CLA was higher in the group that consumed pasture-fed meat and milk. Unfortunately, no information was provided on the diet fed to pasture-raised and grain-fed cattle. Taken together, these data suggest the lipid content of animal products may affect lipoprotein profiles of consumers, and that pasture-raised meat and milk may have greater anti-inflammatory properties compared to feedlot-finished animals. However, evidence is too sparse to make definitive claims and further clinical nutrition trials are needed.
Suitability and Scalability of Pasture-Based Livestock Systems
Grass-fed beef represents 4% of the US beef market (Cheung and McMahon, 2017) and organic milk represent 5.5% of the US dairy market (Gerdes, 2019). Organic milk that is truly from pasture-raised cattle may represent only 0.5%, though that market is growing rapidly and achieved a 58% increase in sales in 2018 (Gerdes, 2019). While concentrated animal feeding operations (CAFOs) are the predominant model in the US, in countries such as New Zealand, Australia as well as some European, South-American, and African nations the finishing model may be increasingly pasture-based. Especially in the US and Western countries the issue is complicated by confusion over the many labels—such as “organic,” “grass-fed,” “pasture-raised,” and “free-range”—that consumers generally associate with healthier products. For example, organic does not necessarily mean animals were raised and finished on pasture. According to the USDA, the requirement is for cattle to have free access to certified-organic pasture for the entire grazing season (at least 120 days), while only 30% of the cattle's diet needs to come from pasture (USDA, 2016a). In the recent past, US farmers could make a good living by switching to organic production, but organic dairying is becoming a victim of its own success. A handful of large scale “organic” dairies—which now feed thousands of cows a diet of organic grain and stored forages with limited access to pasture—produce more milk than all organic, grass-fed dairy farms in Wisconsin combined (Whoriskey, 2017). To further complicate the matter, grass-fed also does not necessarily mean animals were raised on pasture without confinement (Provenza et al., 2019). Even the term “grass-fed” can mean animals were fed grass pellets in a feedlot-type production model or were grazing monoculture grasses. As we have described, this does not result in similar phytochemical richness and favorable fatty acid profiles compared to animals raised on pasture with access to a wide variety of different grasses, forbs, and shrubs. The uncertainty about product quality may be the result of a change in the USDA Agricultural Marketing Service (AMS) regulation of standards for “grass-fed.” While the claim “grass-fed” can still be made through the USDA, AMS discontinued the verification of the applicant's programs to the Standard in 2016. To truly know whether animals were raised on pasture, consumers would have to rely on third-party verification (e.g., “100% Pasture-Raised,” “Global Animal Partnership 5-Step® Animal Welfare Rating,” “Regenerative Organic CertifiedTM″ etc.), establish contact with local farmers, and/or use Internet resources to gain insight into production practices of farmers.
With increased conversion to pasture-based beef production systems in the US, some suggest that domestic beef consumption will have to be reduced by about 40% due to lack of land (Hayek and Garrett, 2018). This estimate includes feeding roughage on pasture and is based on the current status quo of continuous grazing practices. To help meet needs, most people in high-income countries, such as the US and Europe, can reduce red meat consumption with no harm to their health, and likely even improve their health when diet quality would increase as a result (Fogelholm et al., 2015; Grosso et al., 2017; Guasch-Ferré et al., 2019). However, there are several opportunities by which improved management practices can increase the carrying capacity of livestock in pasture-based models, while enhancing ecosystem function. For example, grazing management practices that use ecological principles can increase the carrying capacity of livestock by 50–70% compared to continuous (largely unmanaged) grazing (Jacobo et al., 2006; Jakoby et al., 2015; Wang et al., 2016; Teague and Kreuter, 2020). This is a key point in discussions on whether pasture-based systems can support demand and productivity (Gerrish, 2004). Managed grazing positively influences plant-soil interactions, including plant root exudation and mycorrhiza (Gianinazzi et al., 2010), by recycling nutrients in feces and urine back into the soil through the actions of microorganism and small soil animals (e.g., earthworms and dung beetles) (Holter, 1983; Nichols et al., 2008; Menta and Remelli, 2020), which improves soil health and ecosystem function. Moreover, nutrient-rich soils and plant biomass have potential to improve the health of animals and humans by increasing the phytonutrient density (e.g., terpenoids, phenols, and other anti-oxidants) of pasture-raised meat and milk. In support of the soil-plant-animal-human health connection is that grazing nutrient-rich soils can positively impact the mineral content of grass-fed beef (Leheska et al., 2008). Additional long-term ecosystem benefits from agroecological grazing systems include increased plant and animal biodiversity, carbon sequestration, wildlife habitat, water infiltration and retention, and last but not least, improved resiliency and profitability for farmers through reduction of input cost (Gerrish, 2004; Meuret and Provenza, 2015; Teague and Kreuter, 2020). For a further discussion on soil health and ecosystem function in the context of agroecological grazing systems we refer the reader to the recent work of Teague and Kreuter (2020).
There is also potential for increased carrying capacity from multi-species grazing with little dietary overlap. For instance, integrating cattle with sheep, goats, and pigs and/or potentially other feed-conversion-efficient herbivores such as ducks, geese, and rabbits can improve animal productivity compared to grazing single species (Dumont et al., 2020; Martin et al., 2020) This synergy is achieved because different species exploit different ecological niches and one species can increase resource availability for another species (Walker, 1994; Anderson et al., 2012; Dumont et al., 2020). This would mean that we would have to diversify our meat and milk intake to include products from other livestock such as goats, sheep, bison, duck, geese, and rabbits to name a few. It is noteworthy that consumption of products from many of these livestock is already common practice on other parts of the world or are increasing rapidly in the US (e.g., bison consumption).
Greater diversification of livestock can allow for more efficient use of the resources provided by a particular ecosystem. For example, goats and sheep readily eat species of forbs, shrubs and trees that large herbivores like cattle and bison often avoid, while larger herbivores can better utilize lower quality forage compared to small herbivores such as sheep and goats (Steuter and Hidinger, 1999; Fraser et al., 2014; Cuchillo-Hilario et al., 2018; Martin et al., 2020). These examples illustrate that selection of animals that most effectively use, and more importantly, conserve the natural resource base in a given geographical location should be a key consideration to improve the efficiency and scalability of pasture-based livestock systems. For example, in the US, 53% of all red meat consumed is from beef, 46% is from pork, while <1% comes from other herbivores (STATISTA, 2019). Limiting consumption to only two to three species conflicts with herbivore diversity found in natural ecosystems, and arguably the level of diversity that is desired in agroecologically appropriate livestock systems.
Differences in phytochemicals and fatty acids between animal species further illustrates the importance of livestock diversification—different species provide different nutrients in the human diet. For example, β-carotene and lutein is lower in the meat and milk of goats and sheep compared to bovines (Yang et al., 1992; Lucas et al., 2008). On the other hand, retinol (vitamin A) concentrations are 2-fold higher in milk and meat of ovines compared to bovines (Martin et al., 2004; Darwish et al., 2016), owing to a higher efficiency of converting carotenoids into retinol in ovines (Yang and Tume, 1993). The ω-3 fatty acid content of milk and meat from goats is also 1- to 2-fold higher compared to cow's milk and meat, while cow's milk contains 1.5- to 2-fold higher concentrations of CLA compared to goat's milk (Yang et al., 1992; Lucas et al., 2008). Finally, lamb meat contains 1.5- to 2-fold higher concentrations of CLA compared to beef (Schmid et al., 2006).
Strategic supplementation of livestock on pasture with industrial by-products, inedible to humans (Sunvold et al., 1991; Macdonald et al., 2007), also has potential to increase the carrying capacity of pasture-based grazing systems and mitigate potential welfare issues associated with feedlots, such as reduced ability to express natural behavior and self-selection of diets (Atwood et al., 2001; Villalba and Manteca, 2019). For example, feeding limited amounts of phytochemically-rich fruit and vegetable by-products such as leaves, pomace, peels, rinds, pulp, seeds, and stems (Sruamsiri, 2007; Wadhwa and Bakshi, 2013; Salami et al., 2019) to livestock on pasture can potentially mitigate some nutritional deficits, decrease environmental impacts, and reduce the of risk of overgrazing and land unavailability, while enhancing the phytochemical richness of meat and milk (Provenza et al., 2003, 2019; Salami et al., 2019).
To improve the health and fertility of soils, many farmers in North and South America, Europe, and elsewhere are adding cover crops into rotations with cash crops such as wheat, corn, and soybeans on millions of hectares of land. Cover crops can be grasses or broadleaf plants—ideally mixtures of both—and are often rich in phytochemicals. They can be grown in the fall after cash crops are harvested or grown through the entire growing season. However, there are seed and seeding costs for growing cover crops, and a good way for farmers to reclaim these expenses is to graze them with livestock (Bergtold et al., 2019). Grazing not only helps justify the costs of growing cover crops, but cover crops increase carrying capacity by providing feedstuffs to livestock and reducing negative environmental externalities such as soil erosion and chemical runoff associated with both crop and livestock farming (Fisher et al., 2012; Bergtold et al., 2019). These ecosystem services are achieved by nutrient recycling, reduced need for pesticide application, and by strengthening soil-plant-herbivory interactions to achieve synergy between agricultural production and environmental quality (Lemaire et al., 2014). Therefore, cover crop grazing provides further potential to increase land and forage available to pasture-based livestock production systems, while providing important agroecological benefits.
Strategies that integrate multi-species grazing, mixed crop-livestock systems, and/or phytochemically rich by-product feeding should not be viewed as “silver bullet” approaches to climate change or to meet an ever-growing demand for red meat. However, practices that promote good land stewardship and effective use of resources should be incentivized to sustain and improve the natural resource base upon which agriculture depends—in turn, benefiting the presence of health-promoting compounds in meat and milk from productive soils and vegetation.
We do not expect one paradigm (pasture-based grazing systems) to simply replace the other (feedlot-fed systems). That is not how shifts occurred historically. However, as paradigms gain momentum based on mounting evidence in their favor (e.g., the potential of managed grazing to increase the health of soils, plants, animals, and humans), new combinations of practices emerge. Eventually those practices come to better suit the ideas and the interests of various stakeholders (e.g., consumers, farmers, institutions, and industry). Given the environmental and human health concerns regarding the high-input feedlot model, the livestock industry will arguably have to shift toward an increasingly regenerative hybrid in the future. Even within pasture-based systems, there will be a need for a paradigm shift from conventional (e.g., unmanaged systems that risk overgrazing and land degradation) to agroecological practices that mimic processes of natural ecosystems (e.g., adaptive grazing, integrated crop-livestock systems, multi-species grazing, silvopasture systems etc.). If changes are not taken on an industry wide-scale, the livestock industry may be at risk from increasing societal and institutional pressures to adopt policies that will eventually force change (e.g., through loss of market shares and taxation). Concerns about climate change and associations of red meat and dairy with metabolic disease risk have led to rapid expansion of substitutes, which are touted as better than traditional meat and dairy for environmental and human health (Clay et al., 2020; Van Vliet et al., 2020). Moreover, food policy now questions whether red meat should even be consumed as part of environmentally friendly and healthy diets by future generations (Lucas and Horton, 2019; Willett et al., 2019; WBCSD, 2020), despite having nourished Homo Sapiens and its ancestors for the last 1.5 to 3 million years (Gupta, 2016; Andrews and Johnson, 2020).
Conclusion
Plant diversity—and associated phytochemical richness—links animal, human, and environmental health (Provenza et al., 2019). In addition to reducing per capita consumption of meat in industrialized countries (Godfray et al., 2018), human and environmental health can be enhanced through livestock management practices that promote good land stewardship, limit environmental impacts (Wepking et al., 2019; Andrews and Johnson, 2020; Richter et al., 2020; Rosenzweig et al., 2020), and enhance the healthfulness of meat and dairy products (Provenza et al., 2019). While public health recommendations are for reducing red meat consumption to reduce risk of metabolic disease, no consideration is given to animal production practices in these dietary recommendations. That is likely because the literature on animal production systems and human health is limited.
Forage selection by livestock impacts the phytochemical richness of meat and dairy products, with greater botanical diversity resulting in both a wider variety and higher concentrations of health-promoting phytonutrients in meat and milk (Figure 1). Conversely, these phytonutrients are typically undetectable or present in lower concentrations in meat and milk from animals fed grain-based concentrates in confinement. The presence of phytonutrients in animal foods currently remains underappreciated, and is virtually unheard-of in discussions of nutritional differences between pasture-raised (grass-fed) and feedlot-finished (grain-fed) meat and milk, which have focused myopically on omega-3 fatty acids and CLA (Provenza et al., 2019). For this reason, is it often stated that little to no differences exist between grass-fed or grain-fed meat and milk; however, the reductionist focus on fatty acids vastly underestimates the complexity of natural food matrices. It is in the expanded pool of phytonutrients (e.g., terpenoids, phenolics, carotenoids, and tocopherols) where substantial differences between grass-fed and grain-fed meat and milk are observed.
The expanded pool of phytonutrients must be considered in attempts to understand the effects of meat and dairy consumption on human health, such as the dampening of inflammation and oxidative stress linked with cancer, heart disease, and metabolic syndrome—diseases that have been associated with red meat and dairy consumption (Ganmaa et al., 2002; Micha et al., 2012; Zheng et al., 2019; Fraser et al., 2020; Zhong et al., 2020). Though research is sparse, several studies show a potential for anti-inflammatory effects and improved lipoprotein profiles when people consume pasture-raised meat and dairy. How increasing the phytonutrient density of animal foods will modify potential relationships between consumption and metabolic health of consumers needs to be further addressed in clinical studies.
Future research should systematically assess the linkages between phytochemical richness of herbivore diets, the nutrient density of animal products, and their subsequent effects on human metabolic health. This is important as a rich body of agricultural literature exists on the presence of health-promoting phytonutrients—terpenoids, phenols, carotenoids, and tocopherols—in grass-fed meat and milk that have rarely been evaluated in clinical trials for their potential to modulate human health responses to meat and milk consumption. Given the concerns about red meat consumption on human health and the growing interest among producers and consumers in grass-fed meat and dairy products, clinical nutrition studies evaluating cardiometabolic risk biomarkers in response to phytochemically-rich meat and dairy represents a logical next step in the field. Finally, future studies should elucidate critical—and as yet unstudied—linkages between soil health, plant diversity, and the health of livestock and humans. Addressing this research gap will require greater collaborative efforts from the fields of agriculture and medicine.
Author Contributions
SV wrote the first draft of the manuscript. FP and SK provided many suggestions to improve the manuscript. All authors approved the final version of the manuscript.
Conflict of Interest
SV reports a grant from the North Dakota Beef Association to study the health effects of red meat in relation to diet quality. He has not accepted personal honoraria from any organization to prevent undue influence in the eye of the public. FP reports receiving honoraria for his talks about behavior-based management of livestock.
The remaining author declares that the research was conducted in the absence of any commercial or financial relationships that could be construed as a potential conflict of interest.
References
Adams, T. H., Walzem, R. L., Smith, D. R., Tseng, S., and Smith, S. B. (2010). Hamburger high in total, saturated and trans-fatty acids decreases HDL cholesterol and LDL particle diameter, and increases TAG, in mildly hypercholesterolaemic men. Br. J. Nutr. 103, 91–98. doi: 10.1017/S0007114509991516
Adesogan, A. T., Havelaar, A. H., Mckune, S. L., Eilittä, M., and Dahl, G. E. (2019). Animal source foods: sustainability problem or malnutrition and sustainability solution? Perspective matters. Glob. Food Secur. 25:100325. doi: 10.1016/j.gfs.2019.100325
Adler, S. A., Purup, S., Hansen-Møller, J., Thuen, E., and Steinshamn, H. (2015). Phytoestrogens and their metabolites in bulk-tank milk: effects of farm management and season. PLoS ONE 10:e0127187. doi: 10.1371/journal.pone.0127187
Adlof, R. O., Duval, S., and Emken, E. A. (2000). Biosynthesis of conjugated linoleic acid in humans. Lipids 35, 131–135. doi: 10.1007/BF02664761
Agabriel, C., Cornu, A., Journal, C., Sibra, C., Grolier, P., and Martin, B. (2007). Tanker milk variability according to farm feeding practices: vitamins A and E, carotenoids, color, and terpenoids. J. Dairy Sci. 90, 4884–4896. doi: 10.3168/jds.2007-0171
Allard, V., Soussana, J. F., Falcimagne, R., Berbigier, P., Bonnefond, J. M., Ceschia, E., et al. (2007). The role of grazing management for the net biome productivity and greenhouse gas budget (CO2, N2O and CH4) of semi-natural grassland. Agric. Ecosyst. Environ. 121, 47–58. doi: 10.1016/j.agee.2006.12.004
Anderson, D. M., Fredrickson, E. L., and Estell, R. E. (2012). Managing livestock using animal behavior: mixed-species stocking and flerds. Animal 6, 1339–1349. doi: 10.1017/S175173111200016X
Andrews, P., and Johnson, R. J. (2020). Evolutionary basis for the human diet: consequences for human health. J. Intern. Med. 287, 226–237. doi: 10.1111/joim.13011
Apaoblaza, A., Gerrard, S. D., Matarneh, S. K., Wicks, J. C., Kirkpatrick, L., England, E. M., et al. (2020). Muscle from grass- and grain-fed cattle differs energetically. Meat Sci. 161:107996. doi: 10.1016/j.meatsci.2019.107996
Arnaud, M. (2016). “Occurrence in plants and in vitro, animal and human metabolism of chlorogenic acids,” in Green Coffee Bean Extract in Human Health, eds D. Bagchi, H. Moriyama, and A. Swaroop (Boca Raton, FL: CRC Press), 47–88. doi: 10.1201/9781315371153-5
Arnett, D. K., Blumenthal, R. S., Albert, M. A., Buroker, A. B., Goldberger, Z. D., Hahn, E. J., et al. (2019). 2019 ACC/AHA Guideline on the Primary Prevention of Cardiovascular Disease: a Report of the American College of Cardiology/American Heart Association Task Force on Clinical Practice Guidelines. J. Am. Coll. Cardiol. 74, e177–e232. doi: 10.1161/CIR.0000000000000678
Aro, A., Mannisto, S., Salminen, I., Ovaskainen, M. L., Kataja, V., and Uusitupa, M. (2000). Inverse association between dietary and serum conjugated linoleic acid and risk of breast cancer in postmenopausal women. Nutr. Cancer 38, 151–157. doi: 10.1207/S15327914NC382_2
Arya, F., Egger, S., Colquhoun, D., Sullivan, D., Pal, S., and Egger, G. (2010). Differences in postprandial inflammatory responses to a 'modern' v. traditional meat meal: a preliminary study. Br. J. Nutr. 104, 724–728. doi: 10.1017/S0007114510001042
Atwood, S. B., Provenza, F. D., Wiedmeier, R. D., and Banner, R. E. (2001). Influence of free-choice vs mixed-ration diets on food intake and performance of fattening calves. J. Anim. Sci. 79, 3034–3040. doi: 10.2527/2001.79123034x
Aune, D., Lau, R., Chan, D., Vieira, R., Greenwood, D., Kampman, E., et al. (2012). Dairy products and colorectal cancer risk: a systematic review and meta-analysis of cohort studies. Ann. Oncol. 23, 37–45. doi: 10.1093/annonc/mdr269
Baars, T. (2019). “Chapter 4 - regulations and production of raw milk,” in Raw Milk, eds L. A. Nero and A. F. De Carvalho (Academic Press), 65–89. doi: 10.1016/B978-0-12-810530-6.00004-3
Bailey, D. W., Gross, J. E., Laca, E. A., Rittenhouse, L. R., Coughenour, M. B., Swift, D. M., et al. (1996). Mechanisms that result in large herbivore grazing distribution patterns. Rangeland Ecol. Manage. 49, 386–400. doi: 10.2307/4002919
Barabási, A.-L., Menichetti, G., and Loscalzo, J. (2020). The unmapped chemical complexity of our diet. Nat. Food 1, 33–37. doi: 10.1038/s43016-019-0005-1
Benbrook, C. M., Davis, D. R., Heins, B. J., Latif, M. A., Leifert, C., Peterman, L., et al. (2018). Enhancing the fatty acid profile of milk through forage-based rations, with nutrition modeling of diet outcomes. Food Sci. Nutr. 6, 681–700. doi: 10.1002/fsn3.610
Bergamo, P., Fedele, E., Iannibelli, L., and Marzillo, G. (2003). Fat-soluble vitamin contents and fatty acid composition in organic and conventional Italian dairy products. Food Chem. 82, 625–631. doi: 10.1016/S0308-8146(03)00036-0
Bergtold, J. S., Ramsey, S., Maddy, L., and Williams, J. R. (2019). A review of economic considerations for cover crops as a conservation practice. Renew. Agric. Food Syst. 34, 62–76. doi: 10.1017/S1742170517000278
Besle, J., Lamaison, J., Pradel, P., Fraisse, D., Viala, D., and Martin, B. (2004). Les flavonoïdes, des fourrages au lait. Renc. Rech. Rum. 11, 67–70.
Besle, J. M., Viala, D., Martin, B., Pradel, P., Meunier, B., Berdagué, J., et al. (2010). Ultraviolet-absorbing compounds in milk are related to forage polyphenols. J. Dairy Sci. 93, 2846–2856. doi: 10.3168/jds.2009-2939
Bessa, R. J. B., Santos-Silva, J., Ribeiro, J., and Portugal, A. V. (2000). Reticulo-rumen biohydrogenation and the enrichment of ruminant edible products with linoleic acid conjugated isomers. Livest. Prod. Sci. 63, 201–211. doi: 10.1016/S0301-6226(99)00117-7
Børge, G. I., Sandberg, E., Oyaas, J., and Abrahamsen, R. K. (2016). Variation of terpenes in milk and cultured cream from Norwegian alpine rangeland-fed and in-door fed cows. Food Chem. 199, 195–202. doi: 10.1016/j.foodchem.2015.11.098
Bouvard, V., Loomis, D., Guyton, K. Z., Grosse, Y., Ghissassi, F. E., Benbrahim-Tallaa, L., et al. (2015). Carcinogenicity of consumption of red and processed meat. Lancet Oncol. 16, 1599–1600. doi: 10.1016/S1470-2045(15)00444-1
Brenna, J. T. (2002). Efficiency of conversion of alpha-linolenic acid to long chain n-3 fatty acids in man. Curr. Opin. Clin. Nutr. Metab. Care 5, 127–132. doi: 10.1097/00075197-200203000-00002
Brown, A. W., Trenkle, A. H., and Beitz, D. C. (2011). Diets high in conjugated linoleic acid from pasture-fed cattle did not alter markers of health in young women. Nutr. Res. 31, 33–41. doi: 10.1016/j.nutres.2010.12.003
Brown, G. (2018). Dirt to Soil: One Family's Journey into Regenerative Agriculture. White River Junction, VT: Chelsea Green Publishing.
Bugaud, C., Buchin, S., Hauwuy, A., and Coulon, J.-B. (2001). Relationships between flavour and chemical composition of Abondance cheese derived from different types of pastures. Lait 81, 757–773. doi: 10.1051/lait:2001162
Burkepile, D. E., and Parker, J. D. (2017). Recent advances in plant-herbivore interactions. F1000Res. 6:119. doi: 10.12688/f1000research.10313.1
Butler, G., Nielsen, J. H., Slots, T., Seal, C., Eyre, M. D., Sanderson, R., et al. (2008). Fatty acid and fat-soluble antioxidant concentrations in milk from high- and low-input conventional and organic systems: seasonal variation. J. Sci. Food Agric. 88, 1431–1441. doi: 10.1002/jsfa.3235
Cabiddu, A., Delgadillo-Puga, C., Decandia, M., and Molle, Giovanni (2019). Extensive ruminant production systems and milk quality with emphasis on unsaturated fatty acids, volatile compounds, antioxidant protection degree and phenol content. Animals 9:771. doi: 10.3390/ani9100771
Calder, P. C. (2010). Omega-3 fatty acids and inflammatory processes. Nutrients 2, 355–374. doi: 10.3390/nu2030355
Calderón, F., Chauveau-Duriot, B., Pradel, P., Martin, B., Graulet, B., Doreau, M., et al. (2007). Variations in carotenoids, vitamins A and E, and color in cow's plasma and milk following a shift from hay diet to diets containing increasing levels of carotenoids and vitamin E. J. Dairy Sci. 90, 5651–5664. doi: 10.3168/jds.2007-0264
Cardona, F., Andrés-Lacueva, C., Tulipani, S., Tinahones, F. J., and Queipo-Ortuño, M. I. (2013). Benefits of polyphenols on gut microbiota and implications in human health. J. Nutr. Biochem. 24, 1415–1422. doi: 10.1016/j.jnutbio.2013.05.001
Carrillo, J. A., He, Y., Li, Y., Liu, J., Erdman, R. A., Sonstegard, T. S., et al. (2016). Integrated metabolomic and transcriptome analyses reveal finishing forage affects metabolic pathways related to beef quality and animal welfare. Sci. Rep. 6:25948. doi: 10.1038/srep25948
Catanese, F., Obelar, M., Villalba, J. J., and Distel, R. A. (2013). The importance of diet choice on stress-related responses by lambs. Appl. Anim. Behav. Sci. 148, 37–45. doi: 10.1016/j.applanim.2013.07.005
Chadwick, M., Trewin, H., Gawthrop, F., and Wagstaff, C. (2013). Sesquiterpenoids lactones: benefits to plants and people. Int. J. Mol. Sci. 14, 12780–12805. doi: 10.3390/ijms140612780
Chan, D. S., Lau, R., Aune, D., Vieira, R., Greenwood, D. C., Kampman, E., et al. (2011). Red and processed meat and colorectal cancer incidence: meta-analysis of prospective studies. PLoS ONE 6:e20456. doi: 10.1371/journal.pone.0020456
Chen, C., Han, L., Yu, Q.-L., and Li, R.-R. (2015). Color stability and antioxidant capacity of yak meat as affected by feeding with pasture or grain. Can. J. Anim. Sci. 95, 189–195. doi: 10.4141/cjas-2014-129
Cheung, R., and McMahon, P. (2017). Back to Grass: The Market Potential for U.S. Grassfed Beef . Pocantico Hills, NY: Stone Barns Center for Food and Agriculture.
Chion, A. R., Tabacco, E., Giaccone, D., Peiretti, P. G., Battelli, G., and Borreani, G. (2010). Variation of fatty acid and terpene profiles in mountain milk and “Toma piemontese” cheese as affected by diet composition in different seasons. Food Chem. 121, 393–399. doi: 10.1016/j.foodchem.2009.12.048
Ciccone, M. M., Cortese, F., Gesualdo, M., Carbonara, S., Zito, A., Ricci, G., et al. (2013). Dietary intake of carotenoids and their antioxidant and anti-inflammatory effects in cardiovascular care. Mediators Inflamm. 2013:782137. doi: 10.1155/2013/782137
Clarke, H. J., Griffin, C., Rai, D. K., O'Callaghan, T. F., O'Sullivan, M. G., Kerry, J. P., et al. (2020). Dietary compounds influencing the sensorial, volatile and phytochemical properties of bovine milk. Molecules 25, 1–28. doi: 10.3390/molecules25010026
Clay, N., Sexton, A. E., Garnett, T., and Lorimer, J. (2020). Palatable disruption: the politics of plant milk. Agric. Hum. Values 37, 945–962. doi: 10.1007/s10460-020-10022-y
Coleman, D. C., and Whitman, W. B. (2005). Linking species richness, biodiversity and ecosystem function in soil systems. Pedobiologia 49, 479–497. doi: 10.1016/j.pedobi.2005.05.006
Coppa, M., Chassaing, C., Sibra, C., Cornu, A., Verbič, J., Golecký, J., et al. (2019). Forage system is the key driver of mountain milk specificity. J. Dairy Sci. 102, 10483–10499. doi: 10.3168/jds.2019-16726
Craig, W. J. (1999). Health-promoting properties of common herbs. Am. J. Clin. Nutr. 70, 491s−499s. doi: 10.1093/ajcn/70.3.491s
Cuchillo-Hilario, M., Delgadillo-Puga, C., Navarro-Ocaña, A., and Romo, F. (2009). Antioxidant activity, bioactive polyphenols in Mexican goats' milk cheeses on summer grazing. J. Dairy Res. 77, 20–26. doi: 10.1017/S0022029909990161
Cuchillo-Hilario, M., Wrage-Mönnig, N., and Isselstein, J. (2018). Forage selectivity by cattle and sheep co-grazing swards differing in plant species diversity. Grass Forage Sci. 73, 320–329. doi: 10.1111/gfs.12339
Dadáková, E., Pelikánová, T., and Kalač, P. (2011). Concentration of biologically active polyamines in meat and liver of sheep and lambs after slaughter and their changes in mutton during storage and cooking. Meat Sci. 87, 119–124. doi: 10.1016/j.meatsci.2010.09.009
Daley, C. A., Abbott, A., Doyle, P. S., Nader, G. A., and Larson, S. (2010). A review of fatty acid profiles and antioxidant content in grass-fed and grain-fed beef. Nutr. J. 9:10. doi: 10.1186/1475-2891-9-10
Dalli, J., Colas, R. A., and Serhan, C. N. (2013). Novel n-3 immunoresolvents: structures and actions. Sci. Rep. 3:1940. doi: 10.1038/srep01940
D'Amico, D. J., and Donnelly, C. W. (2010). Microbiological quality of raw milk used for small-scale artisan cheese production in Vermont: effect of farm characteristics and practices. J. Dairy Sci. 93, 134–147. doi: 10.3168/jds.2009-2426
Darwish, W. S., Ikenaka, Y., Morshdy, A. E., Eldesoky, K. I., Nakayama, S., Mizukawa, H., et al. (2016). β-carotene and retinol contents in the meat of herbivorous ungulates with a special reference to their public health importance. J. Vet. Med. Sci. 78, 351–354. doi: 10.1292/jvms.15-0287
Das Gupta, S., and Suh, N. (2016). Tocopherols in cancer: an update. Mol. Nutr. Food Res. 60, 1354–1363. doi: 10.1002/mnfr.201500847
De Faccio Carvalho, P. C., Anghinoni, I., De Moraes, A., De Souza, E. D., Sulc, R. M., Lang, C. R., et al. (2010). Managing grazing animals to achieve nutrient cycling and soil improvement in no-till integrated systems. Nutr. Cycl. Agroecosyst. 88, 259–273. doi: 10.1007/s10705-010-9360-x
De Feo, V., Quaranta, E., Fedele, V., Claps, S., Rubino, R., and Pizza, C. (2006). Flavonoids and terpenoids in goat milk in relation to forage intake. Ital. J. Food Sci. 18, 85–92.
De Gavelle, E., Huneau, J.-F., and Mariotti, F. (2018). Patterns of protein food intake are associated with nutrient adequacy in the general french adult population. Nutrients 10, 1–14. doi: 10.3390/nu10020226
Delcourt, C., Cristol, J.-P., Tessier, F., Léger, C. L., Descomps, B., Papoz, L., et al. (1999). Age-related macular degeneration and antioxidant status in the POLA study. Arch. Ophthalmol. 117, 1384–1390. doi: 10.1001/archopht.117.10.1384
Delgadillo-Puga, C., Cuchillo-Hilario, M., León-Ortiz, L., Ramírez-Rodríguez, A., Cabiddu, A., Navarro-Ocaña, A., et al. (2019). Goats' feeding supplementation with acacia farnesiana pods and their relationship with milk composition: fatty acids, polyphenols, and antioxidant activity. Animals 9:515. doi: 10.3390/ani9080515
Demment, M. W., and Van Soest, P. J. (1985). A nutritional explanation for body-size patterns of ruminant and nonruminant herbivores. Am. Nat. 125, 641–672. doi: 10.1086/284369
Deng, G.-F., Lin, X., Xu, X.-R., Gao, L.-L., Xie, J.-F., and Li, H.-B. (2013). Antioxidant capacities and total phenolic contents of 56 vegetables. J. Funct. Foods 5, 260–266. doi: 10.1016/j.jff.2012.10.015
Descalzo, A. M., Insani, E. M., Biolatto, A., Sancho, A. M., García, P. T., Pensel, N. A., et al. (2005). Influence of pasture or grain-based diets supplemented with vitamin E on antioxidant/oxidative balance of Argentine beef. Meat Sci. 70, 35–44. doi: 10.1016/j.meatsci.2004.11.018
Descalzo, A. M., Rossetti, L., Grigioni, G., Irurueta, M., Sancho, A. M., Carrete, J., et al. (2007). Antioxidant status and odour profile in fresh beef from pasture or grain-fed cattle. Meat Sci. 75, 299–307. doi: 10.1016/j.meatsci.2006.07.015
Dhalla, N. S., Temsah, R. M., and Netticadan, T. (2000). Role of oxidative stress in cardiovascular diseases. J. Hypertens. 18, 655–673. doi: 10.1097/00004872-200018060-00002
Dhiman, T. R., Anand, G. R., Satter, L. D., and Pariza, M. W. (1999). Conjugated linoleic acid content of milk from cows fed different diets. J. Dairy Sci. 82, 2146–2156. doi: 10.3168/jds.S0022-0302(99)75458-5
Di Trana, A., Bonanno, A., Cecchini, S., Giorgio, D., Di Grigoli, A., and Claps, S. (2015). Effects of Sulla forage (Sulla coronarium L.) on the oxidative status and milk polyphenol content in goats. J. Dairy Sci. 98, 37–46. doi: 10.3168/jds.2014-8414
Dian, P. H. M., Chauveau-Duriot, B., Prado, I. N., and Prache, S. (2007). A dose-response study relating the concentration of carotenoid pigments in blood and reflectance spectrum characteristics of fat to carotenoid intake level in sheep1. J. Anim. Sci. 85, 3054–3061. doi: 10.2527/jas.2006-477
Ding, M., Li, J., Qi, L., Ellervik, C., Zhang, X., Manson, J. E., et al. (2019). Associations of dairy intake with risk of mortality in women and men: three prospective cohort studies. BMJ 367:l6204. doi: 10.1136/bmj.l6204
Ding, S., Jiang, H., and Fang, J. (2018). Regulation of immune function by polyphenols. J. Immun. Res. 2018:1264074. doi: 10.1155/2018/1264074
Drouillard, J. S. (2018). Current situation and future trends for beef production in the United States of America - A review. Asian Aust. J. Anim. 31, 1007–1016. doi: 10.5713/ajas.18.0428
Duckett, S. K., Neel, J. P. S., Fontenot, J. P., and Clapham, W. M. (2009). Effects of winter stocker growth rate and finishing system on: III. Tissue proximate, fatty acid, vitamin, and cholesterol content1. J. Anim. Sci. 87, 2961–2970. doi: 10.2527/jas.2009-1850
Dumont, B., Puillet, L., Martin, G., Savietto, D., Aubin, J., Ingrand, S., et al. (2020). Incorporating diversity into animal production systems can increase their performance and strengthen their resilience. Front. Sustain. Food Syst. 4:109. doi: 10.3389/fsufs.2020.00109
Dunne, P. G., Monahan, F. J., O'Mara, F. P., and Moloney, A. P. (2009). Colour of bovine subcutaneous adipose tissue: a review of contributory factors, associations with carcass and meat quality and its potential utility in authentication of dietary history. Meat Sci. 81, 28–45. doi: 10.1016/j.meatsci.2008.06.013
Eisenhauer, B., Natoli, S., Liew, G., and Flood, V. M. (2017). Lutein and zeaxanthin-food sources, bioavailability and dietary variety in age-related macular degeneration protection. Nutrients 9:120. doi: 10.3390/nu9020120
Engelken, J., Carnero-Montoro, E., Pybus, M., Andrews, G. K., Lalueza-Fox, C., Comas, D., et al. (2014). Extreme population differences in the human zinc transporter ZIP4 (SLC39A4) are explained by positive selection in Sub-Saharan Africa. PLoS Genet. 10:e1004128. doi: 10.1371/journal.pgen.1004128
Eshel, G., Stainier, P., Shepon, A., and Swaminathan, A. (2019). Environmentally optimal, nutritionally sound, protein and energy conserving plant based alternatives to U.S. Meat. Sci. Rep. 9:10345. doi: 10.1038/s41598-019-50289-8
Esposito, K., and Giugliano, D. (2006). Diet and inflammation: a link to metabolic and cardiovascular diseases. Eur. Heart J. 27, 15–20. doi: 10.1093/eurheartj/ehi605
Etkin, N. L. (2000). Eating on the Wild Side: The Pharmacologic, Ecologic and Social Implications of Using Noncultigens. Tucson, AZ: University of Arizona Press.
FAO (2009). The State of Food and Agriculture. Livestock in the Balance. Rome: Food and Agriculture Organization. Available online at: http://www.fao.org/3/a-i0680e.pdf (accessed September 21, 2020).
FAO (2010). The Second Report on the State of the World's Plant Genetic Resources for Food and Agriculture. Rome: Food and Agriculture Organization. Available online at: http://www.fao.org/3/i1500e/i1500e00.htm (accessed September 14, 2020).
FAO (2020). Suite of Food Security Indicators. Rome: Food and Agricultural Organization. Available online at: http://www.fao.org/faostat/en/#data/FS (accessed October 9, 2020).
FAOSTAT (2020). Land Use Module. Rome: Food and Agricultural Organization. Available online at: http://www.fao.org/faostat/en/#data/RL/visualize (accessed October 10, 2020).
Fedele, V., Pizzillo, M., Salvatore, C., and Cifuni, G. (2007). Effect of types of forage on terpenes content and profile in goat milk. Options Méd. Sér. A Sémin. Méd. 74, 19–24.
Fischer Boel, M. (2009). Comission Regulation (EC) No 977/2009. Official Journal of the European Union L274/19, 1–6.
Fisher, J., Tozer, P., and Abrecht, D. (2012). Livestock in no-till cropping systems- A story of trade-offs. Anim. Prod. Sci. 52, 197–214. doi: 10.1071/AN11123
Fogelholm, M., Kanerva, N., and Männistö, S. (2015). Association between red and processed meat consumption and chronic diseases: the confounding role of other dietary factors. Eur. J. Clin. Nutr. 69, 1060–1065. doi: 10.1038/ejcn.2015.63
Fraser, G. E., Jaceldo-Siegl, K., Orlich, M., Mashchak, A., Sirirat, R., and Knutsen, S. (2020). Dairy, soy, and risk of breast cancer: those confounded milks. Int. J. Epidemiol. 49, 1–12. doi: 10.1093/ije/dyaa007
Fraser, M. D., Moorby, J. M., Vale, J. E., and Evans, D. M. (2014). Mixed grazing systems benefit both upland biodiversity and livestock production. PLoS ONE 9:e89054. doi: 10.1371/journal.pone.0089054
Ganmaa, D., Li, X.-M., Wang, J., Qin, L.-Q., Wang, P.-Y., and Sato, A. (2002). Incidence and mortality of testicular and prostatic cancers in relation to world dietary practices. Int. J. Cancer 98, 262–267. doi: 10.1002/ijc.10185
Gatellier, P., Mercier, Y., and Renerre, M. (2004). Effect of diet finishing mode (pasture or mixed diet) on antioxidant status of Charolais bovine meat. Meat Sci. 67, 385–394. doi: 10.1016/j.meatsci.2003.11.009
Gerdes, S. (2019). “Grass-fed dairy sector small, but growing rapidly,” in Dairy Foods. Available online at: https://www.dairyfoods.com/articles/93994-grass-fed-dairy-sector-small-but-growing-rapidly (accessed January 3, 2020).
Gerrish, J. (2004). Management-Intensive Grazing: The Grassroots of Grass Farming. Ridgeland, MS: Green Park Press.
Giacco, F., and Brownlee, M. (2010). Oxidative stress and diabetic complications. Circ. Res. 107, 1058–1070. doi: 10.1161/CIRCRESAHA.110.223545
Giacometti, F., Bonilauri, P., Serraino, A., Peli, A., Amatiste, S., Arrigoni, N., et al. (2013). Four-year monitoring of foodborne pathogens in raw milk sold by vending machines in Italy. J. Food Prot. 76, 1902–1907. doi: 10.4315/0362-028X.JFP-13-213
Gianinazzi, S., Gollotte, A., Binet, M. N., Van Tuinen, D., Redecker, D., and Wipf, D. (2010). Agroecology: the key role of arbuscular mycorrhizas in ecosystem services. Mycorrhiza 20, 519–530. doi: 10.1007/s00572-010-0333-3
Gilmore, L. A., Walzem, R. L., Crouse, S. F., Smith, D. R., Adams, T. H., Vaidyanathan, V., et al. (2011). Consumption of high-oleic acid ground beef increases HDL-cholesterol concentration but both high- and low-oleic acid ground beef decrease HDL particle diameter in normocholesterolemic men. J. Nutr. 141, 1188–1194. doi: 10.3945/jn.110.136085
Godfray, H. C. J., Aveyard, P., Garnett, T., Hall, J. W., Key, T. J., Lorimer, J., et al. (2018). Meat consumption, health, and the environment. Science 361:eaam5324. doi: 10.1126/science.aam5324
Gonzalez-Calvo, L., Ripoll, G., Molino, F., Calvo, J. H., and Joy, M. (2015). The relationship between muscle alpha-tocopherol concentration and meat oxidation in light lambs fed vitamin E supplements prior to slaughter. J. Sci. Food Agric. 95, 103–110. doi: 10.1002/jsfa.6688
Gregorini, P., Villalba, J. J., Chilibroste, P., and Provenza, F. D. (2017). Grazing management: setting the table, designing the menu and influencing the diner. Anim. Prod. Sci. 57, 1248–1268. doi: 10.1071/AN16637
Grodstein, F., Kang, J. H., Glynn, R. J., Cook, N. R., and Gaziano, J. M. (2007). A randomized trial of beta carotene supplementation and cognitive function in men: the Physicians' Health Study II. Arch. Intern. Med. 167, 2184–2190. doi: 10.1001/archinte.167.20.2184
Grosso, G., Micek, A., Godos, J., Pajak, A., Sciacca, S., Galvano, F., et al. (2017). Health risk factors associated with meat, fruit and vegetable consumption in cohort studies: a comprehensive meta-analysis. PLoS ONE 12:e0183787. doi: 10.1371/journal.pone.0183787
Grundy, S. M., Brewer, H. B. Jr, Cleeman, J. I., Smith, S. C. Jr, and Lenfant, C. (2004). Definition of metabolic syndrome: report of the National Heart, Lung, and Blood Institute/American Heart Association conference on scientific issues related to definition. Circulation 109, 433–438. doi: 10.1161/01.CIR.0000111245.75752.C6
Guasch-Ferré, M., Merino, J., Sun, Q., Fitó, M., and Salas-Salvadó, J. (2017). Dietary polyphenols, Mediterranean diet, prediabetes, and Type 2 diabetes: a narrative review of the evidence. Oxid. Med. Cell. Longev. 2017:6723931. doi: 10.1155/2017/6723931
Guasch-Ferré, M., Satija, A., Blondin, S. A., Janiszewski, M., Emlen, E., O'Connor, L. E., et al. (2019). Meta-analysis of randomized controlled trials of red meat consumption in comparison with various comparison diets on cardiovascular risk factors. Circulation 139, 1828–1845. doi: 10.1161/CIRCULATIONAHA.118.035225
Gunderson, L. H., Holling, C. S., and Light, S. S. (1995). Barriers and Bridges to the Renewal of Ecosystems and Institutions. New York, NY: Columbia University Press.
Guo, J., Astrup, A., Lovegrove, J. A., Gijsbers, L., Givens, D. I., and Soedamah-Muthu, S. S. (2017). Milk and dairy consumption and risk of cardiovascular diseases and all-cause mortality: dose–response meta-analysis of prospective cohort studies. Eur. J. Epidemiol. 32, 269–287. doi: 10.1007/s10654-017-0243-1
Haskell, M. J. (2012). The challenge to reach nutritional adequacy for vitamin A: beta-carotene bioavailability and conversion–evidence in humans. Am. J. Clin. Nutr. 96, 1193s−1203s. doi: 10.3945/ajcn.112.034850
Havemose, M. S., Weisbjerg, M. R., Bredie, W. L. P., and Nielsen, J. H. (2004). Influence of feeding different types of roughage on the oxidative stability of milk. Int. Dairy J. 14, 563–570. doi: 10.1016/j.idairyj.2003.11.005
Hayek, M. N., and Garrett, R. D. (2018). Nationwide shift to grass-fed beef requires larger cattle population. Environ. Res. Lett. 13:084005. doi: 10.1088/1748-9326/aad401
Helzlsouer, K. J., Huang, H.-Y., Alberg, A. J., Hoffman, S., Burke, A., Norkus, E. P., et al. (2000). Association between α-tocopherol, γ-tocopherol, selenium, and subsequent prostate cancer. J. Natl. Cancer Inst. 92, 2018–2023. doi: 10.1093/jnci/92.24.2018
Henning, S., Fajardo-Lira, C., Lee, H., Youssefian, A., Go, V., and Heber, D. (2003). Catechin content of 18 teas and a green tea extract supplement correlates with the antioxidant capacity. Nutr. Cancer 45, 226–235. doi: 10.1207/S15327914NC4502_13
Hoikkala, A., Mustonen, E., Saastamoinen, I., Jokela, T., Taponen, J., Saloniemi, H., et al. (2007). High levels of equol in organic skimmed Finnish cow milk. Mol. Nutr. Food Res. 51, 782–786. doi: 10.1002/mnfr.200600222
Holmer-Jensen, J., Karhu, T., Mortensen, L. S., Pedersen, S. B., Herzig, K. H., and Hermansen, K. (2011). Differential effects of dietary protein sources on postprandial low-grade inflammation after a single high fat meal in obese non-diabetic subjects. Nutr. J. 10:115. doi: 10.1186/1475-2891-10-115
Holter, P. (1983). “Effect of earthworms on the disappearance rate of cattle droppings,” in Earthworm Ecology: From Darwin to Vermiculture, eds J. E. Satchell (Dordrecht: Springer), 49–57. doi: 10.1007/978-94-009-5965-1_5
Huang, D., Ou, B., and Prior, R. L. (2005). The chemistry behind antioxidant capacity assays. J. Agric. Food Chem. 53, 1841–1856. doi: 10.1021/jf030723c
Huang, J., Weinstein Stephanie, J., Yu, K., Männistö, S., and Albanes, D. (2019). Relationship between serum alpha-tocopherol and overall and cause-specific mortality. Circ. Res. 125, 29–40. doi: 10.1161/CIRCRESAHA.119.314944
Ip, C., Banni, S., Angioni, E., Carta, G., McGinley, J., Thompson, H. J., et al. (1999). Conjugated linoleic acid-enriched butter fat alters mammary gland morphogenesis and reduces cancer risk in rats. J. Nutr. 129, 2135–2142. doi: 10.1093/jn/129.12.2135
IPCC (2019). Intergovernmental Panel on Climate Change. Climate Change and Land. Available online at: https://www.ipcc.ch/srccl/ (accessed January 6, 2020).
Jackson, R. L., Greiwe, J. S., and Schwen, R. J. (2011). Emerging evidence of the health benefits of S-equol, an estrogen receptor beta agonist. Nutr. Rev. 69, 432–448. doi: 10.1111/j.1753-4887.2011.00400.x
Jacobo, E. J., Rodríguez, A. M., Bartoloni, N., and Deregibus, V. A. (2006). Rotational grazing effects on rangeland vegetation at a farm scale. Rangeland Ecol. Manage. 59, 249–257. doi: 10.2111/05-129R1.1
Jahreis, G., Fritsche, J., and Steinhart, H. (1997). Conjugated linoleic acid in milk fat: high variation depending on production system. Nutr. Res. 17, 1479–1484. doi: 10.1016/S0271-5317(97)00138-3
Jain, R., Bronkema, S. M., Yakah, W., Rowntree, J. E., Bitler, C. A., and Fenton, J. I. (2020). Seasonal differences exist in the polyunsaturated fatty acid, mineral and antioxidant content of U.S. grass-finished beef. PLoS ONE 15:e0229340. doi: 10.1371/journal.pone.0229340
Jakoby, O., Quaas, M. F., Baumgärtner, S., and Frank, K. (2015). Adapting livestock management to spatio-temporal heterogeneity in semi-arid rangelands. J. Environ. Manage. 162, 179–189. doi: 10.1016/j.jenvman.2015.07.047
Janštová, B., Dračková, M., Dlesková, K., Cupáková, Š., Necidová, L., Navrátilová, P., et al. (2011). Quality of raw milk from a farm with automatic milking system in the Czech Republic. Acta Vet. Brno 80, 207–214. doi: 10.2754/avb201180020207
Johns, T. (1994). Ambivalence to the Palatability Factors in Wild Food Plants. Tucson, AZ: University of Arizona Press.
Johnston, B. C., Zeraatkar, D., Han, M. A., Vernooij, R. W. M., Valli, C., El Dib, R., et al. (2019). Unprocessed red meat and processed meat consumption: dietary guideline recommendations from the Nutritional Recommendations (NutriRECS) consortium. Ann. Intern. Med. 171, 756–764. doi: 10.7326/M19-1621
Kaluza, J., Wolk, A., and Larsson, S. C. (2012). Red meat consumption and risk of stroke: a meta-analysis of prospective studies. Stroke 43, 2556–2560. doi: 10.1161/STROKEAHA.112.663286
Kampa, M., Nifli, A. P., Notas, G., and Castanas, E. (2007). Polyphenols and cancer cell growth. Rev. Physiol. Biochem. Pharmacol. 159, 79–113. doi: 10.1007/112_2006_0702
Kappeler, R., Eichholzer, M., and Rohrmann, S. (2013). Meat consumption and diet quality and mortality in NHANES III. Eur. J. Clin. Nutr. 67, 598–606. doi: 10.1038/ejcn.2013.59
Khan, N., and Mukhtar, H. (2007). Tea polyphenols for health promotion. Life Sci. 81, 519–533. doi: 10.1016/j.lfs.2007.06.011
Khoo, H.-E., Prasad, K. N., Kong, K.-W., Jiang, Y., and Ismail, A. (2011). Carotenoids and their isomers: color pigments in fruits and vegetables. Molecules 16, 1710–1738. doi: 10.3390/molecules16021710
Khurana, S., Venkataraman, K., Hollingsworth, A., Piche, M., and Tai, T. C. (2013). Polyphenols: benefits to the cardiovascular system in health and in aging. Nutrients 5, 3779–3827. doi: 10.3390/nu5103779
Kim, G. H., Kim, J. E., Rhie, S. J., and Yoon, S. (2015). The role of oxidative stress in neurodegenerative diseases. Exp. Neurobiol. 24, 325–340. doi: 10.5607/en.2015.24.4.325
Kim, J. K. (2016). An update on the potential health benefits of carotenes. EXCLI J. 15, 1–4. doi: 10.17179/excli12015-17664
King, M.-F., Matthews, M. A., Rule, D. C., and Field, R. A. (1995). Effect of beef packaging method on volatile compounds developed by oven roasting or microwave cooking. J. Agric. Food Chem. 43, 773–778. doi: 10.1021/jf00051a039
King, M. F., Hamilton, B. L., Matthews, M. A., Rule, D. C., and Field, R. A. (1993). Isolation and identification of volatiles and condensable material in raw beef with supercritical carbon dioxide extraction. J. Agric. Food. Chem. 41, 1974–1981. doi: 10.1021/jf00035a030
Kinsella, L. J. (2007). “Vitamin deficiencies and other nutritional disorders of the nervous system,” in Neurology and Clinical Neuroscience, eds A. H. V. Schapira, E. Byrne, S. Dimauro, R. S. J. Frackowiak, R. T. Johnson, Y. Mizuno, M. A. Samuels, S. D. Silberstein, and Z. K. Wszolek. (Philadelphia. PA: Mosby), 1455–1467. doi: 10.1016/B978-0-323-03354-1.50113-9
Klingelschmidt, J., Milner, A., Khireddine-Medouni, I., Witt, K., Alexopoulos, E. C., Toivanen, S., et al. (2018). Suicide among agricultural, forestry, and fishery workers: a systematic literature review and meta-analysis. Scand. J. Work Environ. Health 44, 3–15. doi: 10.5271/sjweh.3682
Kozová, M., Kalač, P., and Pelikánová, T. (2009). Changes in the content of biologically active polyamines during beef loin storage and cooking. Meat Sci. 81, 607–611. doi: 10.1016/j.meatsci.2008.10.018
Krausman, P. R., Naugle, D. E., Frisina, M. R., Northrup, R., Bleich, V. C., Block, W. M., et al. (2009). Livestock grazing, wildlife habitat, and rangeland values. Rangelands 31, 15–20. doi: 10.2111/1551-501X-31.5.15
Kris-Etherton, P. M., Harris, W. S., and Appel, L. J. (2003). Fish consumption, fish oil, omega-3 fatty acids, and cardiovascular disease. Arterioscler. Thromb. Vasc. Biol. 23, e20–e30. doi: 10.1161/01.ATV.0000038493.65177.94
Kris-Etherton, P. M., Hecker, K. D., Bonanome, A., Coval, S. M., Binkoski, A. E., Hilpert, K. F., et al. (2002). Bioactive compounds in foods: their role in the prevention of cardiovascular disease and cancer. Am. J. Med. 113, 71–88. doi: 10.1016/S0002-9343(01)00995-0
Kroes, B. V., Van Den Berg, A., Van Ufford, H. Q., Van Dijk, H., and Labadie, R. (1992). Anti-inflammatory activity of gallic acid. Planta Med. 58, 499–504. doi: 10.1055/s-2006-961535
Kuhnen, S., Moacyr, J. R., Mayer, J. K., Navarro, B. B., Trevisan, R., Honorato, L. A., et al. (2014). Phenolic content and ferric reducing-antioxidant power of cow's milk produced in different pasture-based production systems in southern Brazil. J. Sci. Food Agric. 94, 3110–3117. doi: 10.1002/jsfa.6654
Kuhnt, K., Kraft, J., Moeckel, P., and Jahreis, G. (2006). Trans-11-18:1 is effectively Delta9-desaturated compared with trans-12-18:1 in humans. Br. J. Nutr. 95, 752–761. doi: 10.1079/BJN20051680
Kumar, S., and Deshmukh, R. (2019). Allied Market Research. Organic Dairy Food and Drinks Market by Type (Organic Milk, Organic Yogurt, Organic Cheese, and Other Organic Dairy Food and Drinks): Global Opportunity Analysis and Industry Forecast, 2019–2026. Available online at: https://www.alliedmarketresearch.com/organic-dairy-food-and-drinks-market (accessed October 10, 2020).
La Terra, S., Marino, V. M., Manenti, M., Licitra, G., and Carpino, S. (2010). Increasing pasture intakes enhances polyunsaturated fatty acids and lipophilic antioxidants in plasma and milk of dairy cows fed total mix ration. Dairy Sci. Technol. 90, 687–698. doi: 10.1051/dst/2010100
Larick, D. K., Hedrick, H. B., Bailey, M. E., Williams, J. E., Hancock, D. L., Garner, G. B., et al. (1987). Flavor constituents of beef as influenced by forage- and grain-feeding. J. Food Sci. 52, 245–251. doi: 10.1111/j.1365-2621.1987.tb06585.x
Larsson, S. C., Bergkvist, L., and Wolk, A. (2005). High-fat dairy food and conjugated linoleic acid intakes in relation to colorectal cancer incidence in the Swedish Mammography Cohort. Am. J. Clin. Nutr. 82, 894–900. doi: 10.1093/ajcn/82.4.894
Leheska, J., Thompson, L., Howe, J., Hentges, E., Boyce, J., Brooks, J., et al. (2008). Effects of conventional and grass-feeding systems on the nutrient composition of beef. J. Anim. Sci. 86, 3575–3585. doi: 10.2527/jas.2007-0565
Lemaire, G., Franzluebbers, A., Carvalho, P. C. D. F., and Dedieu, B. (2014). Integrated crop–livestock systems: strategies to achieve synergy between agricultural production and environmental quality. Agric. Ecosyst. Environ. 190, 4–8. doi: 10.1016/j.agee.2013.08.009
Lewandowska, U., Szewczyk, K., Hrabec, E. B., Janecka, A., and Gorlach, S. (2013). Overview of metabolism and bioavailability enhancement of polyphenols. J. Agric. Food Chem. 61, 12183–12199. doi: 10.1021/jf404439b
Libby, P. (2007). Inflammatory mechanisms: the molecular basis of inflammation and disease. Nutr. Rev. 65, S140–S146. doi: 10.1111/j.1753-4887.2007.tb00352.x
Loker, W. M. (1994). Where's the beef?: incorporating cattle into sustainable agroforestry systems in the Amazon Basin. Agrofor. Syst. 25, 227–241. doi: 10.1007/BF00707462
López-Andrés, P., Luciano, G., Vasta, V., Gibson, T., Scerra, M., Biondi, L., et al. (2013). Antioxidant effects of ryegrass phenolics in lamb liver and plasma. Animal 8, 1–8. doi: 10.1017/S1751731113001821
Lucas, A., Rock, E., Agabriel, C., Chilliard, Y., and Coulon, J. B. (2008). Relationships between animal species (cow versus goat) and some nutritional constituents in raw milk farmhouse cheeses. Small Rumin. Res. 74, 243–248. doi: 10.1016/j.smallrumres.2007.03.011
Lucas, T., and Horton, R. (2019). The 21st-century great food transformation. Lancet 393, 386–387. doi: 10.1016/S0140-6736(18)33179-9
Lund, M. N., Heinonen, M., Baron, C. P., and Estévez, M. (2011). Protein oxidation in muscle foods: a review. Mol. Nutr. Food Res. 55, 83–95. doi: 10.1002/mnfr.201000453
Luo, Y., Wang, B., Liu, C., Su, R., Hou, Y., Yao, D., et al. (2019). Meat quality, fatty acids, volatile compounds, and antioxidant properties of lambs fed pasture versus mixed diet. Food Sci. Nutr. 7, 2796–2805. doi: 10.1002/fsn3.1039
Lushchak, V. I. (2014). Free radicals, reactive oxygen species, oxidative stress and its classification. Chem. Biol. Interact. 224, 164–175. doi: 10.1016/j.cbi.2014.10.016
Lynch, M. P., Faustman, C., Silbart, L. K., Rood, D., and Furr, H. C. (2001). Detection of lipid-derived aldehydes and aldehyde:protein adducts in vitro and in beef. J. Food Sci. 66, 1093–1099. doi: 10.1111/j.1365-2621.2001.tb16087.x
Macdonald, J. C., Klopfenstein, T. J., Erickson, G. E., and Griffin, W. A. (2007). Effects of dried distillers grains and equivalent undegradable intake protein or ether extract on performance and forage intake of heifers grazing smooth bromegrass pastures. J. Anim. Sci. 85, 2614–2624. doi: 10.2527/jas.2006-560
Mangialasche, F., Xu, W., Kivipelto, M., Costanzi, E., Ercolani, S., Pigliautile, M., et al. (2012). Tocopherols and tocotrienols plasma levels are associated with cognitive impairment. Neurobiol. Aging 33, 2282–2290. doi: 10.1016/j.neurobiolaging.2011.11.019
Mariaca, R. G., Berger, T. F. H., Gauch, R., Imhof, M. I., Jeangros, B., and Bosset, J. O. (1997). Occurrence of volatile mono- and sesquiterpenoids in highland and lowland plant species as possible precursors for flavor compounds in milk and dairy products. J. Agric. Food Chem. 45, 4423–4434. doi: 10.1021/jf970216t
Marino, L., and Allen, K. (2017). The psychology of cows. Anim. Behav. Cogn. 4, 474–498. doi: 10.26451/abc.04.04.06.2017
Marino, V. M., Schadt, I., La Terra, S., Manenti, M., Caccamo, M., Licitra, G., et al. (2012). Influence of season and pasture feeding on the content of α-tocopherol and β-carotene in milk from Holstein, Brown Swiss and Modicana cows in Sicily. Dairy Sci. Technol. 92, 501–513. doi: 10.1007/s13594-012-0069-2
Martin, B., Cornu, A., Kondjoyan, N., Ferlay, A., Verdier-Metz, I., Pradel, P., et al. (2005). “Milk indicators for recognizing the types of forages eaten by dairy cows,” in Indicators of Milk and Beef Quality, eds J. Hocquette and S. Gigli (Wageningen, Netherlands), 127.
Martin, B., Fedele, V., Ferlay, A., Grolier, P., Rock, E., Gruffat, D., et al. (2004). “Effects of grass-based diets on the content of micronutrients and fatty acids in bovine and caprine dairy products”, in Land Use Systems in Grassland Dominated Regions. Proceedings of the 20th General Meeting of the European Grassland Federation (Luzern: vdf Hochschulverlag AG an der ETH Zurich), 876–886.
Martin, G., Barth, K., Benoit, M., Brock, C., Destruel, M., Dumont, B., et al. (2020). Potential of multi-species livestock farming to improve the sustainability of livestock farms: a review. Agric. Syst. 181:102821. doi: 10.1016/j.agsy.2020.102821
Massey, C. (2017). Call of the Reed Warbler: A New Agriculture, a New Earth. White River Junction, VT: Chelsea Green Publishing.
Mattiello, S., Caroprese, M., Matteo, C. G., Fortina, R., Martini, A., Martini, M., et al. (2018). Typical dairy products in Africa from local animal resources. Ital J. Anim. 17, 740–754. doi: 10.1080/1828051X.2017.1401910
Maximova, K., Khodayari Moez, E., Dabravolskaj, J., Ferdinands, A. R., Dinu, I., Lo Siou, G., et al. (2020). Co-consumption of vegetables and fruit, whole grains, and fiber reduces the cancer risk of red and processed meat in a large prospective cohort of adults from Alberta's tomorrow project. Nutrients 12, 1–21. doi: 10.3390/nu12082265
McAfee, A. J., McSorley, E. M., Cuskelly, G. J., Fearon, A. M., Moss, B. W., Beattie, J. A., et al. (2011). Red meat from animals offered a grass diet increases plasma and platelet n-3 PUFA in healthy consumers. Br. J. Nutr. 105, 80–89. doi: 10.1017/S0007114510003090
McAnlis, G., McEneny, J., Pearce, J., and Young, I. (1999). Absorption and antioxidant effects of quercetin from onions, in man. Eur. J. Clin. Nutr. 53, 92–96. doi: 10.1038/sj.ejcn.1600682
McDowell, L. R., Williams, S. N., Hidiroglou, N., Njeru, C. A., Hill, G. M., Ochoa, L., et al. (1996). Vitamin E supplementation for the ruminant. Anim. Feed Sci. Technol. 60, 273–296. doi: 10.1016/0377-8401(96)00982-0
Menta, C., and Remelli, S. (2020). Soil health and arthropods: from complex system to worthwhile investigation. Insects 11, 1–12. doi: 10.3390/insects11010054
Merfort, I. (2011). Perspectives on sesquiterpene lactones in inflammation and cancer. Curr. Drug Targets 12, 1560–1573. doi: 10.2174/138945011798109437
Meunier-Goddik, L., and Waite-Cusic, J. (2019). “Chapter 15 - Consumers acceptance of raw milk and its products,” in Raw Milk, eds L. A. Nero and A. F. De Carvalho (London: Academic Press), 311–350. doi: 10.1016/B978-0-12-810530-6.00015-8
Meuret, M., and Provenza, F. D. (2015). When art and science meet: integrating knowledge of French Herders with science of foraging behavior. Rangeland Ecol. Manage. 68, 1–17. doi: 10.1016/j.rama.2014.12.007
Micha, R., Michas, G., and Mozaffarian, D. (2012). Unprocessed red and processed meats and risk of coronary artery disease and type 2 diabetes–an updated review of the evidence. Curr. Atheroscler. Rep. 14, 515–524. doi: 10.1007/s11883-012-0282-8
Miller, E., Kaur, G., Larsen, A., Loh, S. P., Linderborg, K., Weisinger, H. S., et al. (2013). A short-term n-3 DPA supplementation study in humans. Eur. J. Nutr. 52, 895–904. doi: 10.1007/s00394-012-0396-3
Mora, S., Szklo, M., Otvos, J. D., Greenland, P., Psaty, B. M., Goff, D. C. Jr., et al. (2007). LDL particle subclasses, LDL particle size, and carotid atherosclerosis in the Multi-Ethnic Study of Atherosclerosis (MESA). Atherosclerosis 192, 211–217. doi: 10.1016/j.atherosclerosis.2006.05.007
Nardini, M., Cirillo, E., Natella, F., and Scaccini, C. (2002). Absorption of phenolic acids in humans after coffee consumption. J. Agric. Food Chem. 50, 5735–5741. doi: 10.1021/jf0257547
Nichols, E., Spector, S., Louzada, J., Larsen, T., Amezquita, S., Favila, M., et al. (2008). Ecological functions and ecosystem services provided by Scarabaeinae dung beetles. Biol. Conserv. 141, 1461–1474. doi: 10.1016/j.biocon.2008.04.011
Nielsen (2020). Retail Sales Data. Available online at: https://www.nielsen.com/us/en/ (accessed July 13, 2020).
Nishino, H., Murakoshi, M., Tokuda, H., and Satomi, Y. (2009). Cancer prevention by carotenoids. Arch. Biochem. Biophys. 483, 165–168. doi: 10.1016/j.abb.2008.09.011
Nisoli, E., Clementi, E., Carruba, M. O., and Moncada, S. (2007). Defective mitochondrial biogenesis: a hallmark of the high cardiovascular risk in the metabolic syndrome? Circ. Res. 100, 795–806. doi: 10.1161/01.RES.0000259591.97107.6c
Nordhagen, S., Beal, T., and Haddal, L. (2020). The Role of Animal-Source Foods in Healthy, Sustainable, and Equitable Food Systems. Global Alliance for Improved Nutrition (GAIN). doi: 10.36072/dp.5
Norris, L. E., Collene, A. L., Asp, M. L., Hsu, J. C., Liu, L. F., Richardson, J. R., et al. (2009). Comparison of dietary conjugated linoleic acid with safflower oil on body composition in obese postmenopausal women with type 2 diabetes mellitus. Am. J. Clin. Nutr. 90, 468–476. doi: 10.3945/ajcn.2008.27371
Nozière, P., Graulet, B., Lucas, A., Martin, B., Grolier, P., and Doreau, M. (2006). Carotenoids for ruminants: from forages to dairy products. Anim. Feed Sci. Technol. 131, 418–450. doi: 10.1016/j.anifeedsci.2006.06.018
O'Callaghan, T. F., Sugrue, I., Hill, C., Ross, R. P., and Stanton, C. (2019). “Chapter 7 - nutritional aspects of raw milk: a beneficial or hazardous food choice,” in Raw Milk, eds L. A. Nero and A. F. De Carvalho (London: Academic Press), 127–148. doi: 10.1016/B978-0-12-810530-6.00007-9
Omamo, S. W., Diao, X., Wood, S., Chamberlin, J., You, L., Benin, S., et al. (2006). Strategic Priorities for Agricultural Development in Eastern and Central Africa. Intl Food Policy Res Inst. Research Reports 150. Available online at: http://www.ifpri.org/sites/default/files/publications/rr150.pdf
Pan, A., Sun, Q., Bernstein, A. M., Schulze, M. B., Manson, J. E., Willett, W. C., et al. (2011). Red meat consumption and risk of type 2 diabetes: 3 cohorts of US adults and an updated meta-analysis. Am. J. Clin. Nutr. 94, 1088–1096. doi: 10.3945/ajcn.111.018978
Park, J.-Y., Ale, S., Teague, W. R., and Jeong, J. (2017). Evaluating the ranch and watershed scale impacts of using traditional and adaptive multi-paddock grazing on runoff, sediment and nutrient losses in North Texas, USA. Agric. Ecosyst. Environ. 240, 32–44. doi: 10.1016/j.agee.2017.02.004
Pena-Oyarzun, D., Bravo-Sagua, R., Diaz-Vega, A., Aleman, L., Chiong, M., Garcia, L., et al. (2018). Autophagy and oxidative stress in non-communicable diseases: a matter of the inflammatory state? Free Radic. Biol. Med 124, 61–78. doi: 10.1016/j.freeradbiomed.2018.05.084
Phillips, S. M., Fulgoni, V. L. III., Heaney, R. P., Nicklas, T. A., Slavin, J. L., and Weaver, C. M. (2015). Commonly consumed protein foods contribute to nutrient intake, diet quality, and nutrient adequacy. Am. J. Clin. Nutr. 101, 1346s−1352s. doi: 10.3945/ajcn.114.084079
Pilling, D., Bélanger, J., and Hoffmann, I. (2020). Declining biodiversity for food and agriculture needs urgent global action. Nat. Food 1, 144–147. doi: 10.1038/s43016-020-0040-y
Pizzoferrato, L., Manzi, P., Marconi, S., Fedele, V., Claps, S., and Rubino, R. (2007). Degree of antioxidant protection: a parameter to trace the origin and quality of goat's Milk and Cheese. J. Dairy Sci. 90, 4569–4574. doi: 10.3168/jds.2007-0093
Poutaraud, A., Michelot-Antalik, A., and Plantureux, S. (2017). Grasslands: a source of secondary metabolites for livestock health. J. Agric. Food Chem. 65, 6535–6553. doi: 10.1021/acs.jafc.7b00425
Prache, S., Cornu, A., Berdagué, J. L., and Priolo, A. (2005). Traceability of animal feeding diet in the meat and milk of small ruminants. Small Rumin. Res. 59, 157–168. doi: 10.1016/j.smallrumres.2005.05.004
Prache, S., Martin, B., and Coppa, M. (2020). Review: authentication of grass-fed meat and dairy products from cattle and sheep. Animal 14, 854–863. doi: 10.1017/S1751731119002568
Prache, S., Priolo, A., and Grolier, P. (2003). Effect of concentrate finishing on the carotenoid content of perirenal fat in grazing sheep: its significance for discriminating grass-fed, concentrate-fed and concentrate-finished grazing lambs. Anim. Sci. 77, 225–233. doi: 10.1017/S1357729800058963
Priolo, A., Cornu, A., Krogmann, M., Kondjoyan, N., Micol, D., Berdagué, J. L., et al. (2003). Terpenes in lamb fat to trace animal grass feeding. Ital. J. Anim. 2, 581–583.
Provenza, F. (2018). Nourishment: What Animals Can Teach Us About Rediscovering Our Nutritional Wisdom. White River Junction, VT: Chelsea Green Publishing.
Provenza, F., Villalba, J., Haskell, J. W., Macadam, J., Griggs, T., and Wiedmeier, R. (2007). The value to herbivores of plant physical and chemical diversity in time and space. Crop Sci. 47, 382–398. doi: 10.2135/cropsci2006.02.0083
Provenza, F. D. (1996). Acquired aversions as the basis for varied diets of ruminants foraging on rangelands. J. Anim. Sci. 74, 2010–2020. doi: 10.2527/1996.7482010x
Provenza, F. D. (2008). What does it mean to be locally adapted and who cares anyway? J. Anim. Sci. 86, E271–E284. doi: 10.2527/jas.2007-0468
Provenza, F. D., and Balph, D. F. (1990). “Applicability of five diet-selection models to various foraging challenges ruminants encounter,” in Behavioural Mechanisms of Food Selection, ed R. N. Hughes (Berlin; Heidelberg: Springer), 423–460. doi: 10.1007/978-3-642-75118-9_22
Provenza, F. D., Kronberg, S. L., and Gregorini, P. (2019). Is grassfed meat and dairy better for human and environmental health? Front. Nutr. 6:26. doi: 10.3389/fnut.2019.00026
Provenza, F. D., Villalba, J. J., Dziba, L., Atwood, S. B., and Banner, R. E. (2003). Linking herbivore experience, varied diets, and plant biochemical diversity. Small Rumin. Res. 49, 257–274. doi: 10.1016/S0921-4488(03)00143-3
Quiñonez-Flores, C. M., González-Chávez, S. A., Del Río Nájera, D., and Pacheco-Tena, C. (2016). Oxidative stress relevance in the pathogenesis of the rheumatoid arthritis: a systematic review. Biomed Res. Int. 2016:6097417. doi: 10.1155/2016/6097417
Realini, C. E., Duckett, S. K., Brito, G. W., Dalla Rizza, M., and De Mattos, D. (2004). Effect of pasture vs. concentrate feeding with or without antioxidants on carcass characteristics, fatty acid composition, and quality of Uruguayan beef. Meat Sci. 66, 567–577. doi: 10.1016/S0309-1740(03)00160-8
Reuter, S., Gupta, S. C., Chaturvedi, M. M., and Aggarwal, B. B. (2010). Oxidative stress, inflammation, and cancer: how are they linked? Free Radic. Biol. Med. 49, 1603–1616. doi: 10.1016/j.freeradbiomed.2010.09.006
Richter, B. D., Bartak, D., Caldwell, P., Davis, K. F., Debaere, P., Hoekstra, A. Y., et al. (2020). Water scarcity and fish imperilment driven by beef production. Nat. Sustain. 3, 319–328. doi: 10.1038/s41893-020-0483-z
Ritzenthaler, K. L., McGuire, M. K., McGuire, M. A., Shultz, T. D., Koepp, A. E., Luedecke, L. O., et al. (2005). Consumption of conjugated linoleic acid (CLA) from CLA-enriched cheese does not alter milk fat or immunity in lactating women. J. Nutr. 135, 422–430. doi: 10.1093/jn/135.3.422
Rodríguez-García, C., Sánchez-Quesada, C., Toledo, E., Delgado-Rodríguez, M., and Gaforio, J. J. (2019). Naturally lignan-rich foods: a dietary tool for health promotion? Molecules 24:917. doi: 10.3390/molecules24050917
Röhrle, F. T., Moloney, A. P., Osorio, M. T., Luciano, G., Priolo, A., Caplan, P., et al. (2011). Carotenoid, colour and reflectance measurements in bovine adipose tissue to discriminate between beef from different feeding systems. Meat Sci. 88, 347–353. doi: 10.1016/j.meatsci.2011.01.005
Rosenzweig, C., Mbow, C., Barioni, L. G., Benton, T. G., Herrero, M., Krishnapillai, M., et al. (2020). Climate change responses benefit from a global food system approach. Nat. Food 1, 94–97. doi: 10.1038/s43016-020-0031-z
Rosmond, R. (2005). Role of stress in the pathogenesis of the metabolic syndrome. Psychoneuroendocrinology 30, 1–10. doi: 10.1016/j.psyneuen.2004.05.007
Saha, P., Talukdar, A. D., Nath, R., Sarker, S. D., Nahar, L., Sahu, J., et al. (2019). Role of natural phenolics in hepatoprotection: a mechanistic review and analysis of regulatory network of associated genes. Front. Pharmacol. 10:509. doi: 10.3389/fphar.2019.00509
Salado, E., Bretschneider, G., Cuatrin, A., Descalzo, A., and Gagliostro, G. (2018). Productive response of dairy cows fed with different levels of totally mixed ration and pasture. Agric. Sci. 9, 824–851. doi: 10.4236/as.2018.97058
Salami, S. A., Luciano, G., O'grady, M. N., Biondi, L., Newbold, C. J., Kerry, J. P., et al. (2019). Sustainability of feeding plant by-products: a review of the implications for ruminant meat production. Anim. Feed Sci. Technol. 251, 37–55. doi: 10.1016/j.anifeedsci.2019.02.006
Schmid, A., Collomb, M., Sieber, R., and Bee, G. (2006). Conjugated linoleic acid in meat and meat products: a review. Meat Sci. 73, 29–41. doi: 10.1016/j.meatsci.2005.10.010
Schönfeldt, H. C., Hall, N. G., and Smit, L. E. (2012). The need for country specific composition data on milk. Food Res. Int. 47, 207–209. doi: 10.1016/j.foodres.2011.05.018
Schulze, M. B., Manson, J. E., Willett, W. C., and Hu, F. B. (2003). Processed meat intake and incidence of Type 2 diabetes in younger and middle-aged women. Diabetologia 46, 1465–1473. doi: 10.1007/s00125-003-1220-7
Schwingshackl, L., Hoffmann, G., Lampousi, A. M., Knüppel, S., Iqbal, K., Schwedhelm, C., et al. (2017a). Food groups and risk of type 2 diabetes mellitus: a systematic review and meta-analysis of prospective studies. Eur. J. Epidemiol. 32, 363–375. doi: 10.1007/s10654-017-0246-y
Schwingshackl, L., Schwedhelm, C., Hoffmann, G., Lampousi, A. M., Knüppel, S., Iqbal, K., et al. (2017b). Food groups and risk of all-cause mortality: a systematic review and meta-analysis of prospective studies. Am. J. Clin. Nutr. 105, 1462–1473. doi: 10.3945/ajcn.117.153148
Seddon, J. M., Ajani, U. A., Sperduto, R. D., Hiller, R., Blair, N., Burton, T. C., et al. (1994). Dietary carotenoids, vitamins A, C, and E, and advanced age-related macular degeneration. JAMA 272, 1413–1420. doi: 10.1001/jama.1994.03520180037032
Shelef, O., Weisberg, P. J., and Provenza, F. D. (2017). The value of native plants and local production in an era of global agriculture. Front. Plant Sci. 8:2069. doi: 10.3389/fpls.2017.02069
Sluijs, I., Cadier, E., Beulens, J. W., Van Der, A. D., Spijkerman, A. M., and Van Der Schouw, Y. T. (2015). Dietary intake of carotenoids and risk of type 2 diabetes. Nutr. Metab. Cardiovasc. Dis. 25, 376–381. doi: 10.1016/j.numecd.2014.12.008
Sofi, F., Buccioni, A., Cesari, F., Gori, A. M., Minieri, S., Mannini, L., et al. (2010). Effects of a dairy product (pecorino cheese) naturally rich in cis-9, trans-11 conjugated linoleic acid on lipid, inflammatory and haemorheological variables: a dietary intervention study. Nutr. Metab. Cardiovasc. Dis. 20, 117–124. doi: 10.1016/j.numecd.2009.03.004
Sruamsiri, S. (2007). Agricultural wastes as dairy feed in Chiang Mai. Anim. Sci. J. 78, 335–341. doi: 10.1111/j.1740-0929.2007.00445.x
Stanley, P. L., Rowntree, J. E., Beede, D. K., Delonge, M. S., and Hamm, M. W. (2018). Impacts of soil carbon sequestration on life cycle greenhouse gas emissions in Midwestern USA beef finishing systems. Agric. Syst. 162, 249–258. doi: 10.1016/j.agsy.2018.02.003
STATISTA (2019). Per Capita Consumption of Boneless Red Meat in the U.S. by Type 2017. Available online at: https://www.statista.com/statistics/184378/per-capita-consumption-of-red-meat-in-the-us-2009-by-type/ (accessed October 10, 2020).
Steuter, A. A., and Hidinger, L. (1999). Comparative ecology of bison and cattle on mixed-grass prairie. Great Plains Res. 9, 329–342.
Stover, P. J., and Caudill, M. A. (2008). Genetic and epigenetic contributions to human nutrition and health: managing genome-diet interactions. J. Am. Diet. Assoc. 108, 1480–1487. doi: 10.1016/j.jada.2008.06.430
Sunvold, G. D., Cochran, R. C., and Vanzant, E. S. (1991). Evaluation of wheat middlings as a supplement for beef cattle consuming dormant bluestem-range forage. J. Anim. Sci. 69, 3044–3054. doi: 10.2527/1991.6973044x
Tang, G. (2010). Bioconversion of dietary provitamin A carotenoids to vitamin A in humans. Am. J. Clin. Nutr. 91, 1468S−1473S. doi: 10.3945/ajcn.2010.28674G
Tartaglione, J. E., Alves De Lima, A. E., Vainstein, N., Conde, D., Labruna, M. C., Belardi, J., et al. (2017). Effects of consumption of beef from variably fed cattle on anthropometric measurements, serum parameters and fatty acid composition in healthy men and women. J. Hum. Nutr. Food Sci. 5, 1–10.
Teague, R., and Kreuter, U. (2020). Managing grazing to restore soil health, ecosystem function, and ecosystem services. Front. Sustain. Food Syst. 4:534187. doi: 10.3389/fsufs.2020.534187
Teague, W. (2018). Forages and pastures symposium: cover crops in livestock production: whole-system approach: managing grazing to restore soil health and farm livelihoods. J. Anim. Sci. 96, 1519–1530. doi: 10.1093/jas/skx060
USDA (2015). U.S. Department of Health and Human Services and U.S. Department of Agriculture 2015 – 2020 Dietary Guidelines for Americans, 8th Edn. Available online at: https://health.gov/dietaryguidelines/2015/guidelines/ (accessed January 9, 2019).
USDA (2016a). Organic Production and Handling Standards. US Department of Agriculture. Available online at: https://www.ams.usda.gov/sites/default/files/media/OrganicProductionandHandlingStandards.pdf. (accessed July 7, 2019).
USDA (2016b). USDA, Agricultural Research Service. National Nutrient Database for Standard Reference, release 28. Washington, DC: USDA. Available online at: http://www.ars.usda.gov/ba/bhnrc/ndl (accessed January 2, 2020).
Valdivielso, I., De Renobales, M., Aldai, N., and Barron, L. J. R. (2017). Changes in terpenoid composition of milk and cheese from commercial sheep flocks associated with seasonal feeding regimens throughout lactation. J. Dairy Sci. 100, 96–105. doi: 10.3168/jds.2016-11761
Van Der Steeg, W. A., Holme, I., Boekholdt, S. M., Larsen, M. L., Lindahl, C., Stroes, E. S., et al. (2008). High-density lipoprotein cholesterol, high-density lipoprotein particle size, and apolipoprotein A-I: significance for cardiovascular risk: the IDEAL and EPIC-Norfolk studies. J. Am. Coll. Cardiol. 51, 634–642. doi: 10.1016/j.jacc.2007.09.060
Van Vliet, S., Kronberg, S. L., and Provenza, F. D. (2020). Plant-based meats, human health, and climate change. Front. Sustain. Food Syst. 4:128. doi: 10.3389/fsufs.2020.00128
Vauzour, D. (2012). Dietary polyphenols as modulators of brain functions: biological actions and molecular mechanisms underpinning their beneficial effects. Oxid. Med. Cell Longev. 2012:914273. doi: 10.1155/2012/914273
Verma, S., Singh, A., and Mishra, A. (2013). Gallic acid: molecular rival of cancer. Environ. Toxicol. Pharm. 35, 473–485. doi: 10.1016/j.etap.2013.02.011
Verrier, E., Tixier-Boichard, M., Bernigaud, R., and Naves, M. (2005). Conservation and value of local livestock breeds: usefulness of niche products and/or adaptation to specific environments. Anim. Genet. Resour. 36, 21–31. doi: 10.1017/S1014233900005538
Vialloninsta, C., Martin, B., Verdier-Metz, I., Pradel, P., Garel, J.-P., and Coulon, J.-B. (2000). Transfer of monoterpenes and sesquiterpenesfrom forages into milk fat. Lait 80, 635–641. doi: 10.1051/lait:2000150
Villalba, J. J., and Manteca, X. (2019). A case for eustress in grazing animals. Front. Vet. Sci. 6:303. doi: 10.3389/fvets.2019.00303
Villeneuve, M. P., Lebeuf, Y., Gervais, R., Tremblay, G. F., Vuillemard, J. C., Fortin, J., et al. (2013). Milk volatile organic compounds and fatty acid profile in cows fed timothy as hay, pasture, or silage. J. Dairy Sci. 96, 7181–7194. doi: 10.3168/jds.2013-6785
Voutilainen, S., Nurmi, T., Mursu, J., and Rissanen, T. H. (2006). Carotenoids and cardiovascular health. Am. J. Clin. Nutr 83, 1265–1271. doi: 10.1093/ajcn/83.6.1265
Wadhwa, M., and Bakshi, M. (2013). Utilization of Fruit and Vegetable Wastes as Livestock Feed and as Substrates for Generation of Other Value-Added Products. Bangkok: Food and Agriculture Organization of the United Nations, 1–67.
Wang, S., Moustaid-Moussa, N., Chen, L., Mo, H., Shastri, A., Su, R., et al. (2014). Novel insights of dietary polyphenols and obesity. J. Nutr. Biochem. 25, 1–18. doi: 10.1016/j.jnutbio.2013.09.001
Wang, T., Teague, W. R., and Park, S. C. (2016). Evaluation of continuous and multipaddock grazing on vegetation and livestock performance—a modeling approach. Rangeland Ecol. Manage. 69, 457–464. doi: 10.1016/j.rama.2016.07.003
Wang, Y., and Beydoun, M. A. (2009). Meat consumption is associated with obesity and central obesity among US adults. Int. J. Obes. 33, 621–628. doi: 10.1038/ijo.2009.45
WBCSD (2020). World Business Council for Sustainable Development. Food and Agriculture Roadmap – Chapter on Healthy and Sustainable Diets. Available online at: https://www.wbcsd.org/Programs/Food-and-Nature/Food-Land-Use/FReSH/Resources/Food-Agriculture-Roadmap-Chapter-on-Healthy-and-Sustainable-Diets (accessed November 28, 2020).
Wepking, C., Badgley, B., Barrett, J. E., Knowlton, K. F., Lucas, J. M., Minick, K. J., et al. (2019). Prolonged exposure to manure from livestock-administered antibiotics decreases ecosystem carbon-use efficiency and alters nitrogen cycling. Ecol. Lett. 22, 2067–2076. doi: 10.1111/ele.13390
Werner, L. B., Hellgren, L. I., Raff, M., Jensen, S. K., Petersen, R. A., Drachmann, T., et al. (2013). Effects of butter from mountain-pasture grazing cows on risk markers of the metabolic syndrome compared with conventional Danish butter: a randomized controlled study. Lipids Health Dis. 12:99. doi: 10.1186/1476-511X-12-99
Whaley-Connell, A., McCullough, P. A., and Sowers, J. R. (2011). The role of oxidative stress in the metabolic syndrome. Rev. Cardiovasc. Med. 12, 21–29. doi: 10.3909/ricm0555
Whoriskey, P. (2017). Why your ‘organic' milk may not be organic. Washington Post. Available online at: https://www.washingtonpost.com/business/economy/why-your-organic-milk-may-not-be-organic/2017/05/01/708ce5bc-ed76-11e6-9662-6eedf1627882_story.html?utm_term=.dd97210a72fb (accessed January 2, 2020).
Wiggins, S., Kirsten, J., and Llambí, L. (2010). The future of small farms. World Dev. 38, 1341–1348. doi: 10.1016/j.worlddev.2009.06.013
Willett, W., Rockström, J., Loken, B., Springmann, M., Lang, T., Vermeulen, S., et al. (2019). Food in the Anthropocene: the EAT–Lancet Commission on healthy diets from sustainable food systems. Lancet 393, 447–492. doi: 10.1016/S0140-6736(18)31788-4
Yang, A., Larsen, T., and Tume, R. (1992). Carotenoid and retinol concentrations in serum, adipose tissue and liver and carotenoid transport in sheep, goats and cattle. Aust. J. Agric. Res. 43, 1809–1817. doi: 10.1071/AR9921809
Yang, A., and Tume, R. (1993). A comparison of beta-carotene-splitting activity isolated from intestinal mucosa of pasture-grazed sheep, goats and cattle. Biochem. Mol. Biol. Int. 30, 209–217.
Zhang, H., and Tsao, R. (2016). Dietary polyphenols, oxidative stress and antioxidant and anti-inflammatory effects. Curr. Opin. Food Sci. 8, 33–42. doi: 10.1016/j.cofs.2016.02.002
Zhang, S., Won, Y.-K., Ong, C.-N., and Shen, H.-M. (2005). Anti-cancer potential of sesquiterpene lactones: bioactivity and molecular mechanisms. Curr. Med. Chem. Anticancer Agents 5, 239–249. doi: 10.2174/1568011053765976
Zheng, Y., Li, Y., Satija, A., Pan, A., Sotos-Prieto, M., Rimm, E., et al. (2019). Association of changes in red meat consumption with total and cause specific mortality among US women and men: two prospective cohort studies. BMJ 365:l2110. doi: 10.1136/bmj.l2110
Zhong, V. W., Van Horn, L., Greenland, P., Carnethon, M. R., Ning, H., Wilkins, J. T., et al. (2020). Associations of processed meat, unprocessed red meat, poultry, or fish intake with incident cardiovascular disease and all-cause mortality. JAMA Intern. Med. 180, 503–512. doi: 10.1001/jamainternmed.2019.6969
Zhou, Y., Zheng, J., Li, Y., Xu, D. P., Li, S., Chen, Y. M., et al. (2016). Natural polyphenols for prevention and treatment of cancer. Nutrients 8:E515. doi: 10.3390/nu8080515
Keywords: meat, milk, phytochemicals, organic, grass-fed, health, sustainability, nutrition
Citation: van Vliet S, Provenza FD and Kronberg SL (2021) Health-Promoting Phytonutrients Are Higher in Grass-Fed Meat and Milk. Front. Sustain. Food Syst. 4:555426. doi: 10.3389/fsufs.2020.555426
Received: 24 April 2020; Accepted: 14 December 2020;
Published: 01 February 2021.
Edited by:
Agustin Del Prado, Basque Centre for Climate Change, SpainReviewed by:
Sophie Prache, Institut National de la Recherche Agronomique (INRA), FranceLyudmila Petrova Simova-Stoilova, Institute of Plant Physiology and Genetics (BAS), Bulgaria
Copyright © 2021 van Vliet, Provenza and Kronberg. This is an open-access article distributed under the terms of the Creative Commons Attribution License (CC BY). The use, distribution or reproduction in other forums is permitted, provided the original author(s) and the copyright owner(s) are credited and that the original publication in this journal is cited, in accordance with accepted academic practice. No use, distribution or reproduction is permitted which does not comply with these terms.
*Correspondence: Stephan van Vliet, stephan.vanvliet@duke.edu